Target gene
Injection region
Vector
Experimental model
Functional outcome
References
GAD
Substantia nigra
Engineered cells overexpressing GAD65
Kindling
Increased seizure threshold
Thompson et al. [66]
Substantia nigra
Engineered cells overexpressing GAD65
Spontaneous seizure model
Reduced spontaneous seizures
Thompson and Suchomelova [5]
Piriform cortex
Engineered cells overexpressing GAD65
Kindling
Increased seizure threshold
Gernert et al. [67]
Dentate gyrus
Engineered cells overexpressing GAD65
Kindling
Increased seizure threshold
Thompson [68]
Dentate gyrus
Engineered cells overexpressing GAD65
Granule cell stimulation
Increased threshold and shortened duration of hippocampal afterdischarges
Thompson [68]
Substantia nigra
Engineered cells overexpressing GAD67
Repeated systemic KA
Required larger dose of KA and increased latency to reach stage V seizures, reduced number of seizures
Castillo et al. [69]
Substantia nigra
Engineered cells overexpressing GAD67
Spontaneous seizure model
Reduced seizure behaviors and mortality
Castillo et al. [6]
Substantia nigra
Engineered cells overexpressing GAD67
Kindling
Transient anticonvulsant effects, strong tissue reactions (graft rejection, inflammatory response and gliosis)
Nolte et al. [7]
GABAR-α1
Inferior colliculus
AAV-CMV-GABAR-α1 antisense
Inferior colliculus stimulation
Increased seizure duration
Xiao et al. [8]
Inferior colliculus
AAV-CMV-GABAR-α1
Inferior colliculus stimulation
No effect
Xiao et al. [8]
Hippocampus
AAV-GABAR-α4 promoter-GABAR-α1
Pilocarpine-induced status epilepticus
Transiently reduced spontaneous seizures
Raol et al. [10]
NR1
I.c.v.
NR1 antisense oligodeoxynucleotide
Genetically epilepsy-prone rat
Reduced seizure behaviors
Shan et al. [11]
Collicular cortex
AAV-CMV-NR1 antisense
Focal electrical stimulation
Increased seizure threshold
Haberman et al. [12]
Collicular cortex
AAV-TET off-NR1 antisense
Focal electrical stimulation
Decreased seizure threshold
Haberman et al. [12]
Hippocampus
AAV-CBA-NR1 antisense
Intrahippocampal KA
Increased seizure latency decreased total number of seizures, decreased total time in EEG seizures
Kalev-Zylinska et al. [13]
Peroral administration
AAV-CMV-NR1
Systemic KA
Reduced KA-induced seizures and cell death
During et al. [14]
Galanin
Inferior collicular cortex
AAV-TET off-FIB-GAL
Focal electrical stimulation
Increased seizure threshold
Haberman et al. [18]
Hippocampus
AAV-TET off-FIB-GAL
Systemic KA
Reduced KA-induced seizures and cell death
Haberman et al. [18]
Hippocampus
AAV-NSE-GAL
Intrahippocampal KA
Reduced number of seizure episode and seizure duration, no effect on cell death
Lin et al. [19]
Piriform cortex
AAV-CBA-FIB-GAL
Systemic KA
Reduced EEG and behavioral seizures
McCown [20]
Piriform cortex
AAV-CBA-FIB-GAL
Focal electrical stimulation
Increased seizure threshold
McCown [20]
Neuropeptide Y
Hippocampus
AAV-NSE-NPY
I.c.v. KA and intrahippocampal KA
Delayed seizure onset and attenuated EEG and behavioral seizures
Richichi et al. [21]
Hippocampus
AAV-NSE-NPY
Kindling
Increased seizure threshold, delayed rate of kindling and shortened duration of hippocampal afterdischarges
Richichi et al. [21]
Hippocampus
AAV-CBA-NPY
Rapid hippocampal kindling
Less progression to spontaneous seizures and reduced spontaneous seizures
Noe et al. [22]
Hippocampus
AAV-CBA-NPY
Intrahippocampal KA
Reduced EEG seizures
Noe et al. [23]
Hippocampus
AAV-NSE-NPY
Systemic KA
Reduced seizures in wild-type and Y1 receptor knockout mice but not in Y2 receptor knockout mice. Abolished KA-induced mortality in Y1 receptor knockout mice
Lin et al. [24]
Piriform cortex
AAV-CBA-FIB-NPY, AAV-CBA-FIB-NPY13-36
Systemic KA
Reduced and delayed limbic seizures
Foti et al. [25]
Hippocampus
AAV-NSE-NPY
Subcutaneous KA
Delayed onset to first behavioral seizure and status epilepticus
Woldbye et al. [26]
Hippocampus
AAV-NSE-NPY, AAV-NSE-Y2 receptor
Rapid hippocampal kindling
Increased threshold to severe limbic seizures, reduced number of seizures and shortened duration of hippocampal afterdischarges
Woldbye et al. [26]
Hippocampus
AAV-NSE-NPY, AAV-NSE-Y5 receptor
Subcutaneous KA
Delayed onset to first behavioral seizure and shorter seizure duration
Gotzsche et al. [27]
Hippocampus
AAV-NSE-NPY
Behavioral analysis
Slower rate of hippocampal-based spatial discrimination learning
Sorensen et al. [29]
Hippocampus
AAV-NSE-NPY
Electrophysiological analysis
Attenuated long-term potentiation in CA1-subicular synapses
Sorensen et al. [70]
Hippocampus
AAV-NSE-NPY
Rapid kindling
Reduced seizures
Sorensen et al. [30]
Hippocampus
AAV-NSE-Y1
Behavioral analysis
Modest anxiolytic-like effect in OF, EPM; no effect in TST and FST
Olesen et al. [28]
Hippocampus
AAV-NSE-Y1
Systemic KA
Modestly aggravated KA-induced seizures
Olesen et al. [28]
Hippocampus, Amygdala
AAV-NSE-Y5
Behavioral analysis
No effect on anxiety- and depressive-like behaviors, moderate hyperactivity in OF
Olesen et al. [32]
Hippocampus, Amygdala
AAV-NSE-Y5
Systemic KA
No effect
Olesen et al. [32]
Somatostatin
Hippocampus
AAV-SST
Amygdala kindling
Reduced severe seizures
Zafar et al. [38]
Adenosine
Lateral ventricles
Adenosine releasing ADK deficient baby hamster kidney cells (BHK)
Kindling
Reduced EEG and behavioral seizures
Huber et al. [43]
Lateral ventricle
Adenosine releasing ADA deficient fibroblast
Kindling
Reduced EEG and behavioral seizures
Huber et al. [43]
Lateral ventricles
Adenosine releasing ADK−/− mouse embryonic stem cells
Kindling
Reduced EEG and behavioral seizures
Guttinger et al. [44]
Lateral ventricles
Adenosine releasing ADK−/− mouse C2C12 myoblasts
Kindling
Reduced EEG and behavioral seizures
Guttinger et al. [44]
Hippocampus
Adenosine releasing ADK−/− neural precursor cells
Kindling
Marked suppression of kindling development and reduced EEG and behavioral seizures
Li et al. [45]
Hippocampus
Adenosine releasing human mesenchymal stem cells with lentiviral RNAi mediated ADK knockdown
Intraamygdaloid KA
Reduced seizure duration and CA3 neuronal cell loss
Ren et al. [47]
Hippocampus
Adenosine releasing human mesenchymal stem cells with lentiviral RNAi mediated ADK knockdown
Intraamygdaloid KA
Reduced seizures
Li et al. [71]
Hippocampus
AAV8-gfaABC1D-ADK
Naïve wild-type mice
Induced spontaneous seizures
Theofilas et al. [48]
Hippocampus
AAV8-gfaABC1D-ADK antisense
Spontaneous epileptic Adk-tg transgenic mice
Marked reduction of spontaneous seizures
Theofilas et al. [48]
GDNF
Hippocampus
Ad-CMV-GDNF
Systemic KA
Delayed seizure behavior, reduced seizure severity and neuronal cell loss
Yoo et al. [50]
Hippocampus
AAV-CBA-GDNF
Hippocampal kindling
Decreased the number and severityof seizures, increased seizure threshold
Kanter-Schlifke et al. [51]
FGF-2
Hippocampus
Hippocampal fetal cells pretreated with FGF-2
KA induced chronic TLE
Reduced the frequency of spontaneous recurrent motor seizures
Rao et al. [52]
BDNF/NT-3
Hippocampus
Hippocampal fetal cells pretreated with BDNF and NT-3
KA induced chronic TLE
Reduced the frequency of spontaneous recurrent motor seizures
Rao et al. [52]
BDNF/FGF-2
Hippocampus
HSV-HCMV-FGF-2/ICP0-BDNF
Pilocarpine-induced status epilepticus
Reduced spontaneous EEG seizures, hippocampal neuronal cell death, neuroinflammation, mossy fiber sprouting
ASPA
I.c.v.
Ad-CBA-ASPA
Spontaneous epileptic rats
Transient reduction in tonic seizures
Seki et al. [72]
ICP10PK
Intranasal
HSV-ICP10-ICP10PK
Systemic KA
Reduced behavioral seizures; Reduced neuronal loss, formation of reactive oxygen species, astrogliosis and microglia activation
Laing et al. [57]
CCK
I.c.v.
Lipofectin encapsulated pSV-CCK vector
Audiogenic rats
Transient reduction in seizure vulnerability
Zhang et al. [56]
Glut-1 glucose transporter
Hippocampus
HSV-α22-GT
Intrahippocampal KA
Reduction in KA-induced hippocampal neurotoxicity
McLaughlin et al. [58]
Bcl-2
Hippocampus
HSV-α4-Bcl-2
Intrahippocampal KA
Reduction in KA-induced hippocampal neurotoxicity
McLaughlin et al. [58]
HSP72
Hippocampus
HSV-α22-βgal/α4-HSP72
Systemic KA
Reduced hippocampal neuronal cell death
Yenari et al. [59]
Homer1a
Hippocampus
AAV-CBA-Homer 1
Continuous electrical stimulation
Reduced EEG seizures and prevention of SE
Klugmann et al. [60]
Cardiotrophin 1
Hippocampus
Neural stem cells overexpressing CT1
Lithium-pilocarpine-induced status epilepticus
Reduced seizure induced mossy fiber sprouting
Shu et al. [62]
NpHR 2.0
Motor cortex
Lentivirus-Camk2a-NpHR 2.0
Tetanus toxin injection into the motor cortex
Reduced EEG seizures
Wykes et al. [61]
Kv1.1
Motor cortex
Lentivirus-CMV-Kv1.1
Tetanus toxin injection into the motor cortex
Prevented toxin-induced EEG seizures and spontaneous recurrent seizures; progressively suppressed epileptic activity in an established epileptic focus
Wykes et al. [61]
The first studies targeted the most obvious candidate, the GABAergic system, which has a primary inhibitory role on neurotransmission. With the demonstration by Loscher et al. that transplantation of fetal GABAergic neurons into the substantia nigra (SN) conferred significant, albeit transient, seizure suppression in a rat kindling model [4], most of the subsequent studies focused on ex vivo-engineered GABA-producing cells. Transplantation of genetically engineered cells expressing GAD (glutamic acid decarboxylase, the enzyme that catalyzes the synthesis of GABA) into the SN, piriform cortex, and dentate gyrus showed anticonvulsant effects in both kindling and kainic acid seizure models (Table1). Moreover, transplantation of these GAD-overexpressing cells into the SN of spontaneously seizing animals suppressed spontaneous seizures [5, 6]. Despite the promise of therapeutic efficacy, the advance of cell transplantation approach is severely limited by the poor survival of transplanted cells [5]. Recently, strong inflammatory immune responses followed by graft rejection were reported when engineered GAD67-expressing cells were transplanted into the SN of kindled rats [7].
Others have used a different approach, targeting the GABA receptor subunits rather than direct modulation of GABA levels. An initial attempt by Xiao et al. (1997) using AAV2-mediated gene transfer to upregulate or knockdown the GABAR-α1 subunit in the rat inferior colliculus showed increased seizure sensitivity with GABAR-α1 knockdown but no effect with overexpression [8]. Almost a decade later, Raol et al. (2006) designed an AAV2 construct coding for GABAR-α1 subunit under the control of the α4 subunit (GABRA4) promoter, which is upregulated after status epilepticus [9]. Intrahippocampal injection of this vector led to an increased expression of the α1 subunit at 1–2 weeks after status epilepticus and resulted in a marked protection against recurrent spontaneous seizures [10].
Another logical approach for epilepsy gene therapy is to decrease glutamatergic hyperexcitability by manipulating the N-methyl-d-aspartic acid (NMDA) receptors, which are key receptors in excitatory neurotransmission and important for the propagation of seizures. Repeated intracerebroventricular (i.c.v.) injection of an antisense oligodeoxynucleotide to the NMDA receptor subunit NR1 suppressed seizure behaviors in genetically epilepsy-prone rats and protected cortical neurons from excitotoxicity in vitro [11]. In a study by Haberman et al. (2002), AAV-mediated delivery of antisense RNA to NR1 under the control of the cytomegalovirus (CMV) constitutive promoter into the rat inferior colliculus reduced seizure sensitivity. However, an opposite effect was observed if the antisense NR1 expression was driven by a tetracycline-off regulatable promoter [12]. The authors suggested that the divergent effect on seizure sensitivity was likely due to promoter-related tropic differences as the two constructs transduced distinct neuronal populations. Our group has also investigated the functional consequences of modulating NR1 expression levels in the rat hippocampus using AAV-mediated gene transfer. We found that partial knockdown of the NR1 subunit in the dorsal hippocampus increased seizure latency, decreased the total number of seizures, and decreased the total time in electroencephalographic (EEG) seizures in the hippocampal KA model [13]. Using a radically different approach, we also demonstrated that orally administered AAV-NR1 elicited a humoral autoimmune response against the NR1 subunit. These autoantibodies against NR1 were able to cross the blood–brain barrier and conferred strong antiepileptic and neuroprotective activity in rats subjected to the kainate seizure model at 1–5 months following vaccination [14].
A number of neuropeptides have become targets for developing novel antiepileptic therapies over the past decade. These include neuropeptide Y (NPY), galanin, and somatostatin, which have been shown to modulate neuronal excitability and to function as endogenous anticonvulsants [15–17]. Galanin was the first neuropeptide evaluated as a potential candidate for gene therapy approach. In 2003, Haberman and colleagues designed an AAV vector that expressed and constitutively secreted galanin by fusing the fibronectin secretory sequence (FIB) onto galanin. Infusion of this vector into the rat inferior collicular cortex significantly attenuated focal seizure sensitivity, which could be reversed when galanin expression was suppressed by doxycycline acting on the TET-off promoter of this vector construct. Moreover, intrahippocampal delivery of AAV-FIB-galanin (GAL) resulted in protection against KA-induced seizures and cell death [18]. In the same year, our group also reported the anticonvulsive effect of an AAV vector constitutively overexpressing preprogalanin [19]. We demonstrated that AAV-mediated hippocampal overexpression of preprogalanin was long lasting and decreased intrahippocampal KA-induced seizure activity (number of episodes and duration). More recently, McCown (2006) demonstrated that bilateral AAV-FIB-GAL infusion into the rat piriform cortex produced a marked suppression of KA-induced EEG and behavioral seizure activity [20]. In addition, the vector was injected into the piriform cortex after a series of daily electrical stimulations reached a predetermined threshold of seizure activity. Overexpression of galanin significantly elevated seizure threshold in previously kindled rats.
To date, NPY is the most well-studied target for gene therapy for epilepsy. Together with our collaborators, we first demonstrated the anticonvulsive effect of chronic overexpression of NPY in the hippocampus using recombinant AAV1/2, a chimeric vector consisting of a mixture of AAV1 and AAV2 capsid proteins. Compared to AAV2 alone, AAV1/2-NPY induced superior transduction when infused into the hippocampus and dramatically delayed the onset and decreased the occurrence of epileptic seizures from intracerebral (intracerebroventricular or intrahippocampal) KA administration [21]. We also demonstrated that hippocampal NPY overexpression increased seizure threshold, delayed epileptogenesis, and reduced after discharge duration in the hippocampal kindling model [21]. In order to more accurately assess the effect of rAAV-NPY on epileptogenesis in disease state, the vector was evaluated in a chronic model of spontaneous and progressive temporal lobe epilepsy. In this model, recurrent electric stimulation of the hippocampus leads to the development of spontaneous seizures, and the frequency of seizures increases over time. Intrahippocampal delivery of AAV1/2-NPY in rats already subjected to rapid hippocampal kindling leads to a marked decrease in the progression of seizures [22]. Moreover, spontaneous seizure frequency was dramatically reduced in a subgroup of treated animals compared to their preinjection baseline, indicating that AAV-NPY represents a viable treatment option in the epileptic brain [22]. More recently, improved anticonvulsant effects were achieved in the intrahippocampal KA model using AAV serotype 1 vector, which exhibits greater transduction efficiency [23].
Since the antiepileptic potential of NPY gene transfer has been well established, recent studies focused on improving the anticonvulsant effects and evaluating potential physiological side effects to address safety concerns. Importantly, it was demonstrated that different NPY receptor subtypes exert differential effects on seizure modulation [17]. Our study on the effects of AAV-NPY in Y1 and Y2 receptor knockout mice showed that hippocampal NPY overexpression significantly reduced KA-induced seizures in wild-type and Y1 receptor knockout mice, but not in Y2 receptor knockout mice [24]. In addition, AAV-NPY treatment abolished KA-induced mortality in Y1 receptor knockout mice but not in wild-type or Y2 receptor knockout mice. These findings suggest that Y2 receptor mediates anticonvulsant while Y1 receptor mediates proconvulsant effects. Studies by others were also consistent with this observation. Overexpression of the Y2 receptor-preferring peptide fragment NPY13-36 significantly attenuated KA-induced limbic seizures to the same degree as full-length NPY [25]. More recently, Woldbye et al. showed that AAV-mediated hippocampal overexpression of Y2 receptor resulted in substantial anticonvulsant effects in the rapid kindling model and subcutaneous KA model [26]. More importantly, simultaneous overexpression of NPY and Y2 receptor had a more pronounced seizure-suppressant effect [26]. Similarly, it was demonstrated that combined overexpression of Y5 receptor and NPY exerted significantly stronger seizure suppression compared to NPY overexpression alone, although Y5 overexpression alone did not inhibit KA-induced seizures in rats [27]. In contrast, AAV-mediated overexpression of Y1 receptor modestly aggravated KA-induced seizures in mice, consistent with its proposed proconvulsant property [28].
Several studies also assessed the potential physiological side effects of these NPY-targeted gene therapy approaches. Studies by Sorensen et al. (2008; 2009) found that AAV-NPY delivery to the hippocampus of naïve rats leads to a transient delay in hippocampal-based learning and attenuation of long-term potentiation (LTP) in the CA1 [29]. However, it was also demonstrated in a follow-up study that AAV-NPY treatment does not further compromise LTP in kindled animals, which are already suffering from attenuated LTP [30]. Yet other studies reported no behavioral deficits in rats treated with AAV-NPY with no alterations in learning and memory, anxiety, and locomotor activity [23]. In wild-type mice, intrahippocampal AAV-NPY treatment did not cause obvious adverse effects on the general health, motor function, and cognition and even induced a moderate anxiolytic effect [31]. Interestingly, the treatment also increased depressive-like behavior in the tail suspension test in wild-type mice but not in Y1 receptor knockout mice. In a recent study by Olesen and colleagues, AAV-mediated hippocampal Y1 receptor overexpression was associated with modest anxiety-like effect but had no effect on depressive-like behaviors using the tail suspension and forced swim tests [28]. These authors also showed that hippocampal overexpression of Y5 receptors did not produce significant behavioral effects on anxiety or depression but increased activity in the open field test [32]. In summary, there appear to be limited side effects by NPY gene transfer.
Another neuropeptide proposed to be an endogenous antiepileptic is somatostatin [33]. Similar to galanin and NPY, it is highly expressed in brain regions associated with seizures, and its expression and release are regulated by seizures [34, 35]. Somatostatin knockout mice exhibit heightened seizure severity in the systemic KA model with increased mortality [36]. In addition, exogenous application of somatostatin produced a strong though transient seizure suppression effect in a model of self-sustaining status epilepticus [37]. The anticonvulsant property of somatostatin was recently evaluated by a gene therapy approach [38]. AAV-mediated overexpression of somatostatin in the dentate gyrus and CA1 of the hippocampus protected rats from severe limbic seizures in an electrical amygdala kindling model [38].
The inhibitory neuromodulator adenosine has also attracted interest as an endogenous anticonvulsant [39, 40]. Microdialysis study on patients experiencing seizures showed a dramatic increase in external adenosine levels at seizure foci to a concentration that is sufficient to suppress epileptiform activity in vitro [41]. More recently, it was shown that adenosine may restrict the site of epileptogenesis via activation of A1 receptors as mice deficient in this receptor exhibit extensive neuronal cell death and increased fatality compared to wild-type mice following intrahippocampal KA administration [42]. Using an ex vivo gene therapy approach, Boison, Huber, and colleagues engineered baby hamster kidney cells and fibroblasts to release adenosine by inactivating the adenosine-metabolizing enzymes adenosine kinase (ADK) and adenosine deaminase (ADA), respectively [43]. Transplantation of these encapsulated cells into the brain ventricles of electrically kindled rats provided a nearly complete protection from behavioral seizures and suppressed EEG seizures. However, the antiepileptic effect from released adenosine was transient due to the short-term survival of the transplant [43]. In an attempt to increase the survival time of the transplant and achieve long-term seizure suppression, Boison’s group engineered various types of cells for transplantation, all genetically engineered to release adenosine by genetic inactivation of ADK. These include mouse embryonic stem cells [44] and their differentiated neural precursor cells [45], mouse C2C12 myoblasts [46], and human mesenchymal stem cells with lentiviral RNAi-mediated downregulation of ADK [47]. All of these adenosine-releasing transplants demonstrated antiepileptic and neuroprotective properties [44–47]. However, long-term therapeutic potential remains a challenge due to limited viability of the transplant. Most recently, the group tried an in vivo gene therapy approach using an AAV8 vector to modulate ADK expression in astrocytes [48]. ADK-cDNA in sense or antisense orientation driven by an astrocyte-specific gfaABC1D promoter was expressed in the CA3 of wild-type mice or spontaneous epileptic ADK-overexpressing transgenic mice. Hippocampal overexpression of ADK triggered spontaneous seizures in wild-type mice, whereas ADK downregulation almost completely blocked spontaneous seizures in ADK-overexpressing mice [48].
Neurotrophic factors have also been considered as candidates for epilepsy gene therapy because of their involvement in synaptic plasticity and neuroprotection. They have also been shown to play a complex role in epileptogenesis [49]. The effect of hippocampal glial-derived neurotrophic factor (GDNF) overexpression on seizure was first examined by Yoo and colleagues using an adenoviral vector [50]. Compared to control group, the Ad-GDNF-treated rats had delayed behavioral seizures and reduced seizure severity following intraperitoneal KA administration. In addition, these animals had fewer KA-induced apoptotic cells in the hippocampus. Subsequently, Kanter-Schlifke et al. showed that AAV-GDNF injected into the hippocampus prior to kindling reduced the severity and number of generalized seizures [51]. When given post-kindling, AAV-GDNF increased seizure threshold and reduced seizure frequency during the status epilepticus phase [51]. Similarly, intrahippocampal transplantation of hippocampal fetal cell pretreated with fibroblast growth factor-2 (FGF-2) or brain-derived neurotrophic factor (BDNF) with neurotrophin-3 (NT-3) considerably reduced the frequency of recurrent seizures in a model of chronic TLE [52]. Simonato’s group further demonstrated the antiepileptic and neuroprotective potential of BDNF and FGF-2 by using herpes-based vectors to overexpress these neurotrophins in a chronic epilepsy model after the epileptogenic insult (pilocarpine-induced status epilepticus). This treatment reduced the frequency and severity of spontaneous seizures, reduced seizure-related neuronal cell death, attenuated hippocampal neuroinflammation, and prevented mossy fiber sprouting [53–55].
Finally, although not as well studied as the above targets, various candidates have been demonstrated to have anticonvulsive or protective effects in animal seizure models. These are summarized in Table 1 and include the neuropeptide cholecystokinin (CCK) [56], the antiapoptotic gene ICP 10 protein kinase (ICP10PK) [57], GlutI glucose transporter [58], the apoptosis inhibitor Bcl-2 [58], 72-kd heat shock protein (HSP72) [59], Homer 1 [60], the potassium channel Kv1.1 [61], and cardiotrophin-1 (CT1) [62].
A new development in gene therapy for epilepsy is the use of exogenous or engineered molecules to regulate neuronal excitability. In an elegant study by Wykes et al., the authors explored the potential of an optogenetic gene therapy approach by using a lentiviral vector to express the light-sensitive chloride transporter halorhodopsin in the seizure-generating focus. When laser light was delivered via an optic fiber to this region and the halorhodopsin was activated, a decrease in EEG seizure activity was observed [61]. The success of this optogenetic approach is exciting and suggests that a treatment strategy could be developed to abort seizures on demand without disrupting normal brain function. This is a fast-moving field that is catching momentum as more research groups demonstrate the success of this approach to suppress neuronal excitability and interrupt seizures [63–65].
Clinical Translation
As of 2014, none of the above approaches have advanced beyond the preclinical stage. The most developed approach is that of AAV1-NPY. We, funded in part through the biotechnology company, Neurologix Inc., undertook safety studies in nonhuman primates (marmosets) and subsequently submitted a protocol to the NIH Recombinant DNA Advisory Committee (http://webconferences.com/nihoba/ppt/AAVNPY_final.pdf) and the FDA. Unfortunately, Neurologix Inc. did not have the resources to pursue multiple indications and focused on its AAV-GAD gene therapy product for Parkinson’s disease [66, 67]. Hence, the AAV1-NPY approach is now being developed by us in collaboration with an academic group, headed by Dr. Arjune Sen, at the University of Oxford. Commencement of this trial will be dependent on both the United Kingdom Medical Research Council (MRC) funding and regulatory approvals by the Medicines and Healthcare products Regulatory Agency (MHRA) of the UK.
In addition, the AAV-galanin product is being developed by Asklepios BioPharmaceutical Inc. (AskBio), a privately owned North Carolina, USA, based biotechnology company. The AAV-galanin program is currently in the late preclinical stage, and no hard date has been set for clinical trial initiation. Other approaches appear even further behind, but there is a feeling that gene therapy is finally coming of age, and as the pharmaceutical industry embraces this technology, the clinical translation is likely to become far more rapid.
2 Materials
2.1 AAV Vector Production
HEK293 cells
Plasmids
pFdelta6
pNLrepcap1
AAV pAM packaging plasmid
Complete DMEM/10% FBS
1 L Dulbecco’s Modified Eagle Medium (DMEM, Life Technologies).
10 ml sodium pyruvate.
10 ml nonessential amino acids.
100 ml certified heat-inactivated fetal bovine serum.
5 ml penicillin (10,000 U/ml)/streptomycin sulfate (10,000 μg/ml).
Store at 4 °C in the dark for up to 2 months.
IMDM/5 % FBS
1 L Iscove’s Modified Dulbecco’s Medium (IMDM, Life Technologies).
3.024 g sodium hydrogen carbonate.
50 ml certified heat-inactivated fetal bovine serum.
Store at 4 °C in the dark for up to 2 months.
2× HeBS buffer
11.9 g HEPES (50 mM final concentration).
16.4 g NaCl (28 M final concentration).
0.21 g Na2HPO4 (1.5 mM final concentration).
800 ml tissue culture grade distilled water.
Titrate to pH 7.05 with NaOH.
Add water to 1 L.
Filter sterilize through a 0.22 μm nitrocellulose filter.
Aliquot 10 ml into sterile disposable polypropylene tubes and store at −20 °C.
2.5 M CaCl2
18.37 g CaCl2-2H2O.
Make up in 50 ml distilled water.Stay updated, free articles. Join our Telegram channel
Full access? Get Clinical Tree
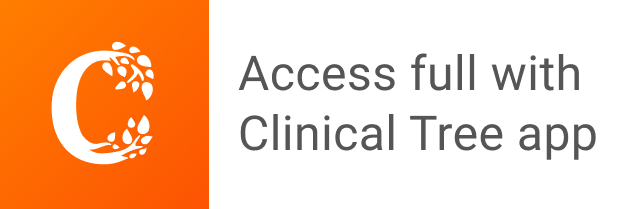