Abstract
Spinal muscular atrophy (SMA) is a devastating neurodegenerative disease that impacts approximately 1:10,000 live births. SMA is caused by deletion or loss-of-function mutations in the Survival Motor Neuron 1 ( SMN1 ) gene. A second gene called SMN2 is alternatively spliced and yields only 10% of full-length SMN protein. As complete loss of SMN protein is embryonic lethal, patients must rely on the low levels of protein derived from SMN2 . SMA is well-suited for viral-based gene therapy as it is a monogenic loss-of-function disorder, the SMN cDNA is relatively small, and the presence of vector-mediated full-length SMN protein will not likely elicit an immune response. Further, SMN2 is found in every patient and provides a strong potential therapeutic target for a myriad of gene therapy strategies. While vector-based gene therapy research has been confounded by a variety of setbacks over the past three decades, recent therapeutic developments have generated a wave of optimism. Based on an extremely solid foundation of compelling preclinical work, SMA gene therapy is now being translated into the clinic and is on the cusp of ushering in a new era of molecular medicine.
Keywords
Spinal muscular atrophy, gene therapy, AAV, scAAV, SMN, clinical trials
Introduction
In the past 20 years, spinal muscular atrophy (SMA) has progressed from a disease of unknown cause to a disease that is generating excitement with multiple ongoing clinical trials for disease-specific therapeutics. This rapid advancement has been beneficial not only to the SMA community, but also to researchers in other fields. SMA is sometimes considered a “model disease” as it is amenable to therapeutic interventions that are adaptable to other disease contexts. Several factors have contributed to the advances in SMA therapy development, including: (1) SMA is monogenic, caused by mutation or deletion of SMN1 ; (2) a nearly identical copy gene, SMN2 is present in all SMA patients; and (3) SMA arises from low levels—not the complete absence—of the survival motor neuron (SMN) protein. The presence of both SMN1 and SMN2 provides two different targets for SMA therapy. Since SMA is caused by loss of SMN1 , viral-based gene replacement of SMN is an attractive strategy. Alternatively, because SMN2 is only functionally distinct from SMN1 in its splicing patterns, antisense oligonucleotides (ASOs) or other splicing modulators designed to promote the proper splicing of SMN2 can also result in the production of full-length SMN protein. While strategies modifying the rate of SMN2 transcription or stability have been tested, this review will focus on the development, optimization, and clinical advancement of viral and ASO-based gene therapies for SMA.
Animal Models
Due to the complex interplay between gene therapy vectors, tissues, and the immune system, developing strategies for gene therapy must be performed on an organismal level. While less complex models such as fruit flies ( Drosophila melanogaster ), nematodes ( Caenorhabditis elegans ), and zebrafish ( Danio rerio ) have been helpful in understanding the molecular roles of SMN, most therapeutic development has been performed in transgenic mouse models. These animal models have been essential as a means of confirming the SMA disease mechanism as well as testing potential therapeutics. SMN2 is unique to humans; thus to truly model the disease, transgenic animal models must be developed in the lab. When animal experiments first began in SMA, the debate on whether SMN1 was the SMA causative gene had been mostly settled. However, after several iterations of genetic manipulation, researchers developed models that displayed characteristic SMA features and further solidified the link between SMN1/2 and SMA. The first mouse SMN ( mSmn ) knockout demonstrated that complete loss of mSmn is embryonic lethal, highlighting the critical importance of SMN in fulfilling basic cellular needs. This experiment failed to create a model of SMA because knockout mice lacked the low levels of SMN protein present in SMA patients that support the viability of the embryo. Integration of the full SMN2 gene into an embryonic lethal mSmn knockout model demonstrated that animals with one to two copies of SMN2 fell into two groups: most of the SMA animals died within 6 hours of birth while 25% lived up to 6 days. This mouse model represents a very severe form of SMA, and only a limited number of interventions can be carried out on such a short-lived animal. In contrast, eight integrated copies of SMN2 yielded healthy pups that lived in excess of 18 months. Another research group designed a mouse model by integrating a larger segment of chromosome 5q13 including SMN2 as well as parts of its neighboring regions. This resulted in mice with variable disease severity: mice termed “type 1” died by day 10, “type 2” died within 2–4 weeks, and “type 3” were long-lived and suffered only from a blunt tail.
To summarize the previous experiments, loss of mSmn is embryonic lethal, the addition of two copies of SMN2 rescued embryonic lethality but the mice die early in life, and eight copies yielded complete rescue. Thus, it was hypothesized that more copies of SMN2 would result in progressively milder symptoms, possibly providing a spectrum of SMA mouse models available to study. Osborne et al . produced an SMN2 allelic series of transgenic mice, replacing the mSmn locus with either 0, 1, 2, 3, 4, 5, 6, or 8 copies of SMN2. However, this allelic series did not demonstrate a strong linear effect between SMN2 and gross disease phenotype. The line receiving two copies of SMN2 was embryonic lethal, whereas addition of three or more copies produced mice that were very healthy with only a moderate behavioral and physiological semblance to SMA even though SMN was restored only to a modest 30–40% of wild-type protein levels. These SMN2 -based models elucidated several concepts: (1) enhancing SMN2 may yield profound results in vivo; (2) increasing SMN levels to ~30–40% of normal may be sufficient for phenotypic improvements; and (3) titrating levels of SMN may be difficult using transgenic means.
A number of additional mouse models have been developed for SMA. The most widely utilized mouse is known as the SMNΔ7 model. This model was created to settle the debate as to whether the truncated SMNΔ7 protein was simply functionally insufficient, or whether it carried a dominant negative effect, exacerbating SMA symptoms. Toward this end, researchers added many copies of the SMNΔ7 cDNA into a severe mSMN −/− , SMN2 +/+ model and found that SMNΔ7 addition extended the model’s lifespan from ~7 days to ~14 days. This lifespan provides adequate time for testing therapeutics before and after symptom onset. Around 8 days of age, the mice experience a sharp decline in muscle strength followed by a very predictable and reproducible death curve, which has been ideal for detecting therapeutic effects. The SMNΔ7 model has been instrumental in advancing the ASO and AAV-based therapies currently in clinical trials. While many other severe, intermediate, and mild SMA models have been developed, they have mostly been used in very early preclinical testing and will not be discussed at length here.
Strategies of Gene Therapy in Spinal Muscular Atrophy
The genetic situation of SMA allows for two possible routes of therapy. Early SMA research recognized that SMN2 is a natural therapeutic target. Thus, researchers have aimed at increasing SMN2 expression, stability, and splicing inclusion of exon 7. Many therapeutic strategies, including bifunctional RNAs, HDAC inhibitors, small molecules, repurposed drugs, aminoglycosides, and ASOs, have been used toward this end with varying degrees of risk and efficacy.
The second route for therapy is based on delivering a replacement for the patient’s missing or nonfunctional SMN1 gene. This is accomplished using a viral vector to deliver the gene of interest so that it can be continually expressed. Safe and effective delivery of viral-based therapeutics can be challenging however, this strategy has quickly developed from a mouse-based preclinical investigation to a clinical trial. Further, delivery of SMN as well as other genes of interest has allowed researchers to probe the pathology and physiology of SMA.
Antisense Oligonucleotides
The genetic context of SMA provides unique opportunities for therapeutic development, many of which are focused on the presence of SMN2 . This gene is an appealing target because it is present in all patients, it is often found in multiple copies, and it is capable of encoding a wild-type SMN protein. This gene fails to rescue the loss of SMN1 because it has the tendency to exclude exon 7 during mRNA splicing; consequently reducing the amount of full-length SMN it produces. Shifting the splicing preferences of the SMN2 pre-mRNA such that it includes exon 7 could, in theory, provide sufficient SMN to replace the function of patients’ missing SMN1 genes. This strategy is highly attractive because modulating the native SMN2 pre-mRNA to produce full-length SMN protein makes it less likely that SMN will accumulate to superphysiologic levels. There is no known toxicity related to overexpression of SMN, but considering the irreversible nature of gene therapies, the utilization of a native SMN transcript provides an additional layer of spatiotemporal regulation that may increase safety. One such therapeutic approach utilizes ASO sequences that target gene-specific splicing modulators.
ASOs are short nucleic acid sequences able to bind a target sequence with a high degree of specificity. When designing specific ASOs for SMA therapeutics, several criteria are considered, including their ability to increase exon retention with high efficiency and specificity, low toxicity, long-lasting resistance to cellular degradation, and high permeability to target cells like motor neurons within the central nervous system (CNS). In SMA, a variety of sequences have been targeted by various chemistries as illustrated in Fig 10.1 .

Alternative splicing plays an important role in gene expression and abnormal splicing has been recognized as a cause of an increasing number of diseases. In SMA, nearly 90% of SMN2-derived transcripts encode a truncated protein that lacks the final coding exon 7. Pre-mRNA splicing of SMN exon 7 is regulated by a variety of factors that bind to pre-mRNA sequences called exonic or intronic splicing enhancers (ESEs) and silencers (ESSs). The role of these enhancer and silencer motifs is to promote or repress the splice-site decision. It has been previously shown that there are a multitude of alternative splice signals within and flanking SMN exon 7.
Several ASOs have been designed utilizing different chemistry backbones and studied in cell and animal models. Two research groups used 2′- O -methyl (2′-OMe) and 2′- O -methoxyethyl (2′-MOE) ASOs targeting the putative ESE abolished by the C to T transition, combined with a tailed splice-factor recruiting ASO. Researchers have also attempted to redirect positive splicing factors to the vicinity of exon 7 using an RNA molecule that can bind to the SMN2 pre-mRNA and recruit splicing factors. These sequences are known as bifunctional RNAs due to the presence of these two functional domains: an RNA sequence that is complementary to the target RNA and inhibits its function (e.g., SMN exon 7, intron 6); and an RNA segment that serves as a sequence-specific binding platform for cellular splicing factors. In SMA, bifunctional RNAs have been designed to inhibit intronic repressors through the antisense sequence and to recruit SR proteins promoting specific ESEs.
Two in vivo studies have examined the efficacy of bifunctional RNAs. In both studies, the bifunctional RNA was able to increase the production of SMN in the CNS. The treated animals exhibited improved motor function, and lifespan was increased by 2–7 days. Nevertheless, due to the unstable chemistry of native RNA, bifunctional RNAs will need to be delivered continuously. Gene therapy vectors expressing bifunctional RNAs driven by a ubiquitous promoter have the potential to overcome this limitation by providing a continuous source of therapeutic RNA.
The mode of action with morpholino-modified oligonucleotides resembles that of ASOs. Morpholino oligonucleotides are synthetic molecules similar in structure to natural nucleic acids, made by substituting morpholine and phosphorodiamidate for ribose and phosphate in the backbone of the molecule. Due to these modifications, morpholinos exhibit greater stability in vivo and do not activate toll-like receptors. Like RNA-based ASOs, morpholinos have been used to block sites on RNA to obstruct cellular processes by binding to its selected target site and hindering access of RNA processing machinery. By blocking sites involved in splicing pre-mRNA, morpholinos can be used to modify normal splicing events. Morpholino-based ASOs targeting different intronic splicing repressors have been shown to be effective at modulating SMN2 splicing in vivo.
Some strategies have utilized ASOs to target the intron 7/exon 8 junction with the goal of reducing recognition of the exon 8 3′-splice-site in favor of the 3′-splice-site of exon 7, increasing exon 7 inclusion. Additionally, employing a bifunctional ASO that recruits negative splice effectors (hnRNP A1/A2) can further facilitate splice suppression. ASO-mediated therapy can also be enhanced by incorporating the ASO into a small nuclear ribonucleic protein-like (snRNP-like) complex. The U7 snRNP is normally involved in the maturation of replication-dependent histone mRNAs, using its guide RNA to target the mRNA for cleavage. This RNA has two regions of interest, one that mediates binding with RNA regulatory proteins called Smith Core proteins (Sm)/Lsm and one that complements the histone pre-mRNA (guide sequence). Altering the Sm/Lsm sequence of the U7 snRNP RNA creates a particle that can bind to the mRNA and competitively inhibit wild-type U7 snRNP activity. When combined with an alternative guide sequence, the new snRNP can inhibit the binding of other RNA binding proteins to a target RNA. For instance, a U7 snRNA containing a sequence complementary to the 3′-splice-site of SMN exon 8 can prevent splicing factors from accessing this portion of the SMN pre-mRNA, increasing the rate of exon 7 inclusion in the mature mRNA.
Though each of these strategies demonstrated the ability to increase exon 7 inclusion in the mature SMN mRNA, vector-based delivery of splicing modulating RNAs has not advanced into animal models. However, treatments employing ASOs have applications, not only for SMA therapy, but also for a number of diseases where altering splicing may be therapeutic, such as Duchenne muscular dystrophy, Huntington’s disease, and myotonic dystrophy.
Utilizing Viruses for Spinal Muscular Atrophy Research
Viral vectors have been utilized for a variety of purposes in SMA research. For SMA therapeutics, viral delivery has provided a means to achieve stable expression of otherwise transient RNA- or protein-based therapies. Researchers have also used viruses to deliver SMN or other genes of interest in order to investigate biological properties of SMA, providing an inexpensive alternative to creating transgenic animals. This approach can provide insight into the spatiotemporal requirements of SMN and may inform SMA treatment development in the future.
Creating Transgenics
Viral-based knockdown of SMN has been able to compensate where transgenic models are unavailable. For instance, preclinical testing and assessment of biomarkers for SMA would benefit from an SMN2 -based large-animal model. Pigs are an attractive candidate for this purpose due to their physiological similarities to humans, and initial studies have shown the feasibility of an SMN2-based swine model. As an alternative approach, self-complementary AAV9 (scAAV9) has been used to deliver shRNAs to knock down the expression of the endogenous SMN. SMN expression in pigs was reduced to a clinically relevant level throughout the CNS, and these animals exhibited SMA-like symptoms such as hindlimb weakness and atrophy. Animals became nonambulant within weeks following delivery of the shRNA vector. This model was subsequently used to examine distribution and efficacy of a scAAV9-SMN vector.
Determining the Spatial Requirements for SMN
The mechanism through which the ubiquitously expressed SMN protein can cause a seemingly motor-neuron-specific disease has been a pressing question within the SMA community. Many have wondered whether this disease is actually specific to motor neurons or whether inadequate SMN levels adversely affect other types of neurons or peripheral tissues. Interestingly, SMN depletion in motor neurons does not cause an overt SMA phenotype, and expression exclusively in motor neurons is not sufficient to rescue the disease in mice. Other transgenic models demonstrated that pan-neuronal restoration of SMN is much more effective than muscle-specific restoration. These findings led to two questions: which other tissue types are affected in SMA and which cell types contribute to the degradation of motor neurons? Viral gene delivery has allowed researchers to probe disease progression in well-established transgenic models. Recently SMN was delivered specifically to astrocytes in the SMNΔ7 and SMN 2B/− models using a scAAV vector delivering SMN under the control of a GFAP promoter. Specificity was ensured by including binding sites for neuronally expressed miRNAs in the 3′-UTR. Astrocyte-specific expression of SMN in the SMNΔ7 model led to a twofold improvement in lifespan. The milder SMN 2B/− animals all survived past 80 days while their untreated counterparts lived for ~35 days. This is one example of how researchers have been able to utilize gene therapy technology to investigate SMA pathogenesis.
Delivering Neuroprotectants
Viral delivery has been used to test therapeutic candidates that address the symptoms of SMA rather than the molecular cause of the disease. Two candidates for this approach have come from the field of amyotrophic lateral sclerosis. Because neurotrophic factors protect motor neurons under stressful conditions, researchers investigated whether these proteins could alter the disease trajectory of SMA. Supplementing SMA mice with the neuroprotectant cardiotrophin 1 ameliorated the phenotypes associated with SMA, though overall extension of life span was modest. Another group utilized insulin-like growth factor-1 to treat a mild SMA mouse model. While this therapy yielded a reduction in motor neuron death, it did not produce a phenotypic improvement.
Replacing SMN1 in Spinal Muscular Atrophy
As SMA is monogenic, vector-mediated gene replacement has been an intriguing area of research for over a decade. The initial proof-of-concept experiments in SMA involved the development of an adenovirus vector that expressed the complete SMN cDNA. In cell-based models, expression of SMN1 restored SMN protein levels, increased the levels of SMN-associated factors such as Gemin proteins, and dramatically increased the nuclear foci referred to as SMN-positive “gems.” However, this vector was not examined in an animal model of disease to test for efficacy.
The first in vivo experiments followed shortly thereafter using a lentiviral platform. Utilizing the natural tropism of the rabies G (Rab-G) protein for neuronal populations, the investigators incorporated a ubiquitously expressed SMN transgene into a Rab-G pseudotyped equine infectious anemia virus. In SMA patient fibroblasts, the vector dramatically elevated levels of SMN and SMN-positive gems. For delivery of the vector to the mouse nervous system, intramuscular (i.m.) injections were performed into the hindlimb gastrocnemius, diaphragm, intercostal, facial, and tongue muscles. The goal of this route was to transduce motor neurons innervating these muscles through retrograde transport, the process responsible for shuttling cellular cargoes from the nerve terminal to the soma. Vector-derived SMN expression was present in spinal cord motor neurons following i.m. injections, and total motor neuron numbers were increased in lenti-SMN-treated animals. These improvements were met with only a mild effect on the gross phenotype. Lenti-SMN extended survival of severe SMA mice from ~13 days to ~18 days while a control virus expressing LacZ extended survival to ~15 days. Despite a fairly small sample size ( n=5 ), each of these improvements was statistically significant. Though the life extension was modest, this work provided a powerful proof-of-concept demonstrating that SMA was a candidate for gene therapy. This allowed the field to optimize vector type, delivery, and timing for SMA therapeutics.
Adeno-associated viral vectors have yielded substantial improvements in disease progression in animal models of SMA. When delivered directly into the CNS via a combination of intracerebroventricular and lumbar intrathecal injections, ssAAV8 and scAAV8 vectors expressing SMN were able to significantly improve the phenotype of a severe model of SMA. ssAAV8 increased median survival to 50 days of age, while scAAV8 increased survival to 150 days and prevented motor neuron loss until close to endpoint.
Intravenous (i.v.) delivery is an attractive injection method due to the minimal risks associated with the procedure as well as its potential to reach the entire body. However, the vasculature of the brain is not permeable to most macromolecules, viruses, and bacteria, preventing them from reaching the brain from the bloodstream. This blood–brain barrier (BBB), therefore, presents a significant obstacle for most i.v. administered gene therapy vectors. One AAV serotype, scAAV9, has been shown to efficiently cross the BBB in multiple species, including mice, adult cats, and nonhuman primates (NHPs), allowing for CNS transduction following intravenous injection ( Fig. 10.2 ). Due to its ability to transit the BBB, many SMA preclinical studies have employed i.v. AAV9. In 2010, researchers intravenously delivered scAAV9 expressing SMN under the control of a ubiquitous (chicken β-actin or CB) promoter. This therapy, scAAV9.CB.SMN, extended the life of SMNΔ7 mice from ~14 days to an unprecedented range of 90–250+ days. Soon after, another group published similar results with a codon-optimized SMN . Improvements in lifespan were associated with increased motor neuron survival and the preservation of innervated neuromuscular junctions ( Fig. 10.3 ).
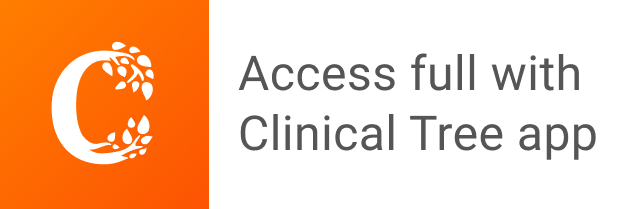