Fig. 1
Schematic of the exon 7 regulation within SMN pre-mRNA and trans-splicing strategy to replace the SMN2 exon 7. (a) SMN pre-mRNA is regulated by cis elements (within intron 6, exon 7, and intron 7) and splicing factors which are involved in the inclusion or exclusion of exon 7. The components of the splicing machinery are shown in yellow. The positively acting splicing factors are shown in green. The negatively acting sequences and splicing factors are shown in red. The C–T transition that creates a splice silencer site in SMN2 transcript is indicated. (b) Cis-splicing of SMN2 pre-mRNA produces two mRNAs due to an alternative splicing event. Trans-splicing strategy utilizes a tsRNA that consists of (1) an antisense domain that binds to intron 6 and blocks the endogenous branch point; (2) the splicing domain with optimal branch point, polypyrimidine tract, and 3’ splice site that carry out the splicing reaction between the SMN2 pre-mRNA and tsRNA; and (3) the SMN1 exon 7 which replaces SMN2 exon 7 following a trans-splicing event. The generation of the trans-spliced RNA is verified by using a primer specific for the M13 sequence located downstream of the exon 7 stop codon. Antisense oligonucleotide (7–11) expressed from the U6 promoter is located downstream of the tsRNA sequence and binds the 3′ splice site of exon 8
1.1.5 SMA Gene Replacement Therapy
First gene replacement strategy in SMA utilized a lentiviral vector expressing full-length human SMN cDNA that was retrogradely transported into motor neurons following multiple injections into a large number of muscles in SMNΔ7 mice [55]. The outcome was more viable motor neurons and a modest extension in life. Recently, the gene therapy approach was taken to an advanced level by using recombinant scAAV serotype 9 expressing a human SMN cDNA. A single injection of 5 × 1011 genomic copies of scAAV9-SMN into temporal vein of SMNΔ7 mice on postnatal day 1 (P1) resulted in a dramatic restoration of motor function and neuromuscular physiology as well as a significant increase in life span up to 250 days [3]. In both studies, the transgene (human SMN cDNA), time of application, and the mouse model (SMNΔ7) were identical. Therefore, the underlying mechanism for this dramatic difference in survival was the robust expression of SMN by scAAV, wide transduction of the CNS by serotype 9, CBA promoter expressing the transgene, and delivery route. We will discuss all of the related gene therapy studies to emphasize the significance of each contributing factor in rescuing the SMA phenotype.
Advantages of scAAV
In contrast to traditional single-stranded AAV (ssAAV), scAAV vector packages a single viral genome possessing both sense and antisense strands of the transgene. After uncoating in the transduced cells, the single-stranded DNA folds into a double-stranded DNA due to its self-complementary nature and forms an appropriate Pol II template for transcription [56]. As a result, scAAV achieves maximal expression levels within 48–72 h post-transduction. Furthermore, in some instances, transduction efficiency of scAAV is 10- to 100-fold higher than ssAAV [57, 58]. An alternative study demonstrated the magnitude of benefits using scAAV versus ssAAV. ssAAV8 expressing human full-length SMN was ICV injected into SMNΔ7 mouse model and extended life to ~50 days [59]. Interestingly, the expression of SMN using scAAV8 through ICV injection extended the survival up to 157 days [59]. Several studies have determined the temporal requirement for SMN induction using transgenic SMA mice and AAV vectors. These studies have concluded that restoration of SMN before the symptomatic stage is necessary for a significant increase in life span [3, 60, 61]. Based on these results, it is not surprising that an efficient rescue of SMA mice has never been achieved with other viral vectors or traditional ssAAV, and thus far, scAAV is the most competent viral vector for SMA gene therapy.
Serotype 9 of AAV
AAV9 was shown to reach the CNS following intravenous administration in neonatal mice and adult cats [62, 63], suggesting that this serotype might be well suited for the delivery of the SMN transgene into SMA patients. The study by Passini et al. [59] which demonstrated the superiority of scAAV to ssAAV also established the advantages of serotype 9 to serotype 8. Mice treated with scAAV8-SMN demonstrated highly improved motor function and neuromuscular physiology compared to their control littermates but died prematurely compared to the mice injected systemically with scAAV9-SMN (157 days vs. 250 days) as reported by Foust et al. [3]. Based on the recent findings of the heart defects in SMA mouse models [64–66], it was suggested that intravenous (IV) injection leads to better survival which is possibly due to its direct cardiac transduction that protects the heart [67]. However, direct comparison of the two injection methods requires all parameters to be identical, and in this case, the AAV serotypes were different. Even though the superiority of AAV9 to AAV8 in global cardiac gene transfer is reported [68, 69] and cardiac malfunction could very well be one of the many possible reasons for premature death of scAAV8-SMN-injected mice, a direct proof that the IV injection is more advantageous than ICV injection was lacking at the time of this publication. Direct comparison of IV and ICV injections in SMA gene therapy was performed in our lab which will be discussed in the following section.
The clinical potential of scAAV9 in neurodegenerative diseases was verified by the ability of scAAV9 to pass the blood-brain barrier and transduce motor neurons when injected into the nonhuman primates [3, 62, 63, 70, 71]. The most extensive study was performed by Bevan et al. in which the CNS accessibility was tested in cynomolgus macaques by IV injection of scAAV9-GFP at various ages up to 3 years of age [72]. They observed transduction of motor neurons in the spinal cord as well as extensive transduction of glial cells in the brain at all the time points tested. Systemic delivery also resulted in the transduction of skeletal muscles along with peripheral organs. They also examined the transgene expression by the directed targeting of the CNS using intrathecal and intracisternal injection of scAAV9-GFP into newborn pigs. This type of delivery may be beneficial for less severe cases which merely require CNS transduction and also reduces the amount of viral vectors required. Both delivery routes using either the cisternal space at the base of the skull or the intrathecal space at L5 led to GFP expression only in the CNS including the dorsal root ganglia, the gray and white matter of the spinal cord including motor neurons at all levels of the spinal cord. This study concluded that the consistency of scAAV9 in transduction of the CNS in a broad spectrum of species and the choice of different delivery routes makes it a suitable vector for neurodegenerative pediatric diseases applied at various ages [72].
Delivery Routes
Some evidence suggests that the rescue of peripheral organs in addition to motor neurons, especially in severe cases of disease, is necessary for the complete rescue of the SMA phenotype [39, 46, 73, 74]. Therefore, the transduction of the peripheral organs which is a definite consequence of the systemic injection plays a beneficial role in SMA gene therapy. Our group aimed to shed light on the suitability of different delivery methods in SMA gene therapy. We examined two injection methods (IV and ICV) directly by utilizing an identical viral vector preparation and titer (2 × 1010 genomic particles), which was approximately ten times less than the dose utilized by Foust et al. [3]. Our results demonstrated a considerable level of increase in strength and weight of scAAV9-SMN-injected mice through ICV delivery than IV delivery [24]. Moreover, our survival analysis indicated that ICV-treated mice displayed fewer premature deaths than IV-treated animals. We concluded that a low titer of scAAV9-SMN can rescue SMA phenotype more efficiently when administered through ICV compared to IV [24]. This may be due to the fact that indirect delivery into the CNS through the circulation system requires a higher dose than a direct delivery through ICV. Even though systemic injection will be more appealing than intrathecal injection in patients, a higher dose required for an efficient treatment may be a limiting factor for this type of delivery. Potential autonomic nervous system (ANS) dysfunction is also perceived as the main cause of partial rescue in IV-injected SMA mice. This possibility is based on the incomplete rescue of cardiac function in IV-injected SMA mice [64, 75] and reports of the ANS defects in SMA patients [76, 77]. Lack of sufficient evidence to prove this suggestion necessitates further studies to reveal the role of ANS malfunction in SMA manifestation and whether the ICV injection is more capable of transducing ANS than IV injection. The potential ability of ICV injection to transduce the peripheral ANS neurons may result from the leakiness of ICV injection at the earlier time points due to the immature state of the blood-brain barrier (Fig. 3).
A very recent publication has demonstrated that the scAAV9 delivered by a single intramuscular (i.m.) injection can be retrogradely transported to motor neurons and also distributed into the bloodstream to transduce peripheral organs in adult and neonatal mice [78]. In this study, the authors injected codon-optimized scAAV9-SMN into both gastrocnemius and triceps muscles of neonatal SMNΔ7 mice and observed increased median survival up to 163 days. Evidently, the high dose of the virus used in this study (5 × 1013 to 1 × 1014 vg/kg) was necessary to achieve the observed level of the rescue using i.m. injection. Regardless, these results prove that a gene delivery system such as scAAV9 has an amazing capability to overcome the limitations of each delivery route and still reach the target cells and additional tissues. Entering into the spinal cord along with the internal organs in a neurodegenerative disease such as SMA which possibly requires transduction of the peripheral organs including the heart is an additive advantage of scAAV9.
Choosing the most efficient delivery route in humans will eliminate the requirement for introducing an enormous amount of the viral vector to achieve a great level of rescue. Lack of SMA models of nonhuman primates may limit our ability to identify the best delivery route for SMA clinical trials. Some labs have aimed to develop an SMA pig model [79], and upon completion, this large animal model may help guide optimizing the delivery paradigms.
Time Point of Application
Interestingly, the developmental stage at which the virus should be introduced to achieve the maximal effect is of great importance. Systemic delivery of scAAV9-SMN into SMNΔ7 model at later time points significantly decreases the therapeutic benefits of the vector. Foust et al. [3] reported that systemic injection of scAAV9-SMN at postnatal day 2 (P2) leads to ~250 days of survival as P1 injection. However, P5 injection led to ~15 days increase in survival and P10 injection had no beneficial effect. The authors concluded that transduction is altered in the CNS depending on the time of injection. P2 injection resulted in high level of transduction in motor neurons, while P10 injection led mostly to glial transduction [3]. Whether this pattern of transduction is responsible for the lack of rescue in P10-injected mice remains to be elucidated. In another study, systemic injection of the scAAV9-GFP (through tail vein) resulted in motor neuron transduction up to 28 % in adult mice and 15 % in adult cats [63]. Since the promoters driving the GFP transgene expression were different in these two studies [chicken β-actin hybrid (CBA) vs. cytomegalovirus (CMV), respectively], the possibility of the promoters functioning differently in neuronal system of adult mice should be considered. An additional question is whether a similar pattern of transduction occurs following ICV injection at delayed time points. Nevertheless, as the likelihood of scAAV9-SMN entering the clinic increases rapidly, determining the underlying mechanism that prevents rescue at delayed time points is essential for patients who will miss the critical time course of therapy.
Viral Titer
In two additional studies, the impact of scAAV9-SMN through temporal facial vein injection was investigated in newborn SMA mice. In the first study, the systemic injection of 1 × 1011 genomic copies of cAAV9 expressing a codon-optimized hSMN cDNA resulted in significant improvements in motor function and an increase in median survival up to 69 days in 80 % of treated animals [22]. This survival rate is significantly less than that reported by Foust et al. [3]. Since in both studies the injections were administered at P1–2, it is hypothesized that the greater increase in survival of the mice reported by Foust et al. is due to a fivefold higher dose of the virus [22]. This speculation may not be justified since the SMN expression in these viruses was driven by two different promoters. Moreover, a second study indicated that systemic injection of 4.5 × 1010 genomic copies of scAAV9-hSMN (also codon-optimized) into newborn SMA mice resulted in survival rate of more than 200 days in 50 % of the treated mice [21]. In this study, 46 % of the injected mice died by 65 days. These findings contradict the possibility that the dose effect is totally responsible for the survival rate and suggest that additional factors in the viral vector play a role in the efficacy of the virus. These factors may include the type of the promoter driving the SMN expression, the method of the virus construction, the purity/quality of the virus, and even variations in the injection methods. According to our study, a low dose (2 × 1010 genomic copies) of scAAV9-SMN is still sufficient to rescue the SMA mice efficiently when delivered through ICV rather than IV. Therefore, the dose of the virus depends greatly on other parameters of the gene therapy to reach a high level of efficiency. In conclusion, appropriate combination of all the parameters of the gene therapy mentioned above is critical to achieve a sufficient level of rescue.
1.2 Summary of Our Research in SMA Gene Therapy
Splicing of SMN exon 7 is regulated by many cis– and trans-acting factors that function positively or negatively leading to either inclusion or exclusion of exon 7 (Fig. 1a) [33, 50]. Our efforts in SMA gene therapy include correction of SMN2 pre-mRNA splicing (increasing the inclusion of exon 7) by direct delivery of the therapeutic RNAs (bifunctional and trans-splicing) as well as modified oligonucleotides into the CNS by ICV injection [44, 80–83]. We have also utilized ssAAV2 to deliver the therapeutic RNAs and scAAV9-SMN to carry out gene replacement therapy [23, 24, 75]. Our research involving the therapeutic RNAs (in the form of plasmid DNA and modified oligonucleotides) has been reviewed in detail previously [50, 53]. Here, we only describe the outcome of our gene therapy research utilizing viral vectors.
1.2.1 Development of ssAAV2 Vectors
Our first attempts to exploit viral vectors involved the construction of traditional ssAAV2 expressing therapeutic RNAs (bifunctional and trans-splicing) which showed great efficacy in increasing the SMN levels in cell-based assays [84, 85]. Our initial trans-splicing RNA (tsRNA) molecule was designed to target intron 6 within the SMN2 pre-mRNA and replace the following 3’ exon (exon 7) with SMN1 exon 7 [85]. This construct (designated pM13) consisted of the following: an SMN intron 6 annealing sequence approximately 130 bp, an optimized heterologous splice site, the SMN1 exon 7 sequence, and a specific sequence (M13 primer) immediately downstream of the native stop codon at the end of exon 7 to allow straightforward detection of the trans-spliced product by RT-PCR (Fig. 1b) [85]. However, the efficiency of the viral vector (ssAAV2) expressing this trans-splicing RNA was negligible in SMA mouse models. Therefore, we optimized the tsRNA by adding an antisense (7–11) to block splicing at the downstream exon (exon 8) to create more opportunities for a trans-splicing event (designated pMU3) (Figs. 1b and 2a) [81, 86]. We concentrated ssAAV2 containing pMU3 by cesium-chloride gradient centrifugation and delivered 1 × 1011 genomic copies of the vector through ICV injection into SMNΔ7 mice at P2. Our goal was to analyze the functionality of the vector to generate a trans-spliced RNA from the SMN2 pre-mRNA in the CNS of the injected SMNΔ7 mice. Using this approach, the 400 bp trans-spliced RNA was detected in the thoracic and cervical spinal cord as well as the muscle of injected animals using exon 4 forward primer (detects human SMN) [87] and M13 reverse primer (Fig. 2b). As expected, the trans-spliced RNA was not detected in untreated animals. The lack of trans-spliced RNA in the brain could be due to the fact that the whole brain homogenates were used for RNA extraction and probably led to a much weaker signal. The lack of trans-splicing in the lumbar spinal cord of injected animal could be due to inadequate amount of tissue that led to the poor quality of RNA. Injection of the pMU3 virus slightly increased the strength of SMNΔ7 mice but had no effect on survival and weight (data not shown). The later attempts involved utilizing a ssAAV2 vector expressing two functional elements: optimized trans-splicing RNA (tsRNA +7–11) and the cDNA of HA-tagged mouse IGF1 under the control of a neuron-specific promoter (pMU4) (Fig. 2a) [82]. Injection of 1 × 1011 genomic copies of the dual vector (pMU4) expressing both tsRNA and IGF-1 did not significantly extend the life span of SMNΔ7 mice (data not shown); however, it increased the body mass and strength (Fig. 2c) [82]. The improvement in phenotype was possibly the result of increased levels of the SMN protein in the lumbar spinal cord of ssAAV2-pMU4-injected SMNΔ7 mice at P12 (Fig. 2d). These results confirmed the capability of ssAAV2 to reach the target tissues, evidenced by increased SMN levels in the lumbar spinal cord. However, the timing of the ssAAV expression which occurs approximately 10 days postinjection made this vector incapable of extending the survival of the SMA mice with a life span of 13–16 days. However, for neurodegenerative diseases in which the mouse model has a life span of 30 days or longer, there is sufficient time for the expression and functionality of ssAAV vectors.
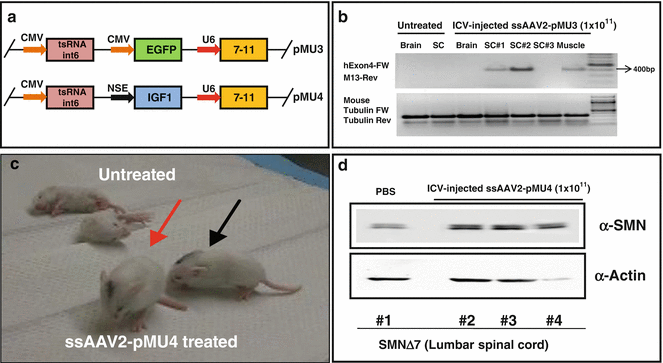
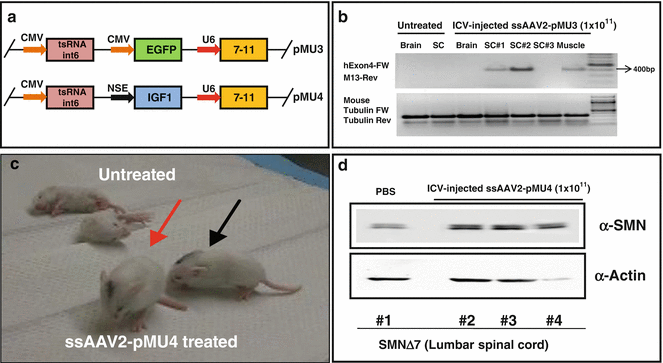
Fig. 2
ssAAV2 vectors expressing trans-splicing RNAs are functional in the spinal cord of ICV-injected SMNΔ7 mice. (a) Map of pMU3 and pMU4 constructs. (b) SMNΔ7 mice were injected at P2 with 1 × 1011 particles of ssAAV2-pMU3. In vivo RT-PCR was performed with RNA extracted from the brain, thoracic (sc#1), cervical (sc#2), lumbar (sc#3) spinal cord, and muscle harvested 10 days postinjection. The 400 bp trans-spliced RNA was detected using exon 4 forward (specific for human SMN) and M13 reverse. RNAs of the untreated mouse were used as negative control, and mouse tubulin primers were used for loading control. (c) SMNΔ7 mice injected with PBS and 1 × 1011 particles of ssAAV2-pMU4 through ICV are shown at 16 days of age. Black and red arrows point to pMU4-injected mice. Several pMU4-injected mice were strong enough to stand on their hind limbs (red arrow). (d) SMNΔ7 mice were injected at P2 with either PBS (mouse #1) or 1 × 1011 particles of ssAAV2-pMU4 (Mouse #2, #3, and #4). The lumbar region of the spinal cord was used for Western blot 12 days postinjection. SMN and actin were detected using monoclonal antibodies
1.2.2 Development of scAAV9 Vectors
Following our results with ssAAV2 and reports of scAAV9 efficacy in motor neurons [62, 63], we prompted to use scAAV9 in our therapeutic interventions with SMA. Initially, we constructed scAAV9-GFP and verified the functionality of the virus by its ability to express GFP in vivo. In addition to test the capability of the virus, we intended to verify the most efficient delivery route for scAAV9 to access the CNS. Therefore, we introduced a very low dose (6 × 109 genomic copies) of scAAV9-GFP via ICV and IV injections into heterozygous (unaffected) SMNΔ7 mice. Western blot using the spinal cord tissue detected GFP expression in both ICV- and IV-injected mice. However, we detected a much greater level of GFP in the spinal cord of the ICV-injected animals than IV-treated mice (Fig. 3a, b). These results verified that a low dose of scAAV9 can access the spinal cord more efficiently through ICV delivery than IV delivery [24]. Since the transduction of peripheral organs (as well as motor neurons) is necessary for the efficient rescue of the SMA phenotype [46, 73, 74] and scAAV9 is capable of reaching the peripheral organs along with the CNS through the circulation system, IV injection was considered to be more sensible and applicable. However, our results in which the rescue of SMA phenotype by ICV injection was more efficient than IV injection [24] led us to assume that the leakiness of ICV injection at P2 (due to immature state of the blood-brain barrier) results in the transduction of the peripheral organs as well. To prove this hypothesis, we ICV injected 1 × 1011 genomic copies of scAAV9-GFP into the heterozygous SMNΔ7 mice, harvested the brain and peripheral organs 5 days postinjection, examined the expression of the GFP in the whole organs, and compared to the organs of the age-matched untreated control. Our results clearly demonstrate that through ICV injection, scAAV9-GFP is capable of transducing the liver, heart, lung, and muscle as well as brain (Fig. 4a–e). The level of GFP expression in the kidney was negligible (data not shown), and a very insignificant level of autoflorescence was captured in all the organs of untreated control mice except the liver (Fig. 4). Based on these results, the superiority of the ICV delivery to IV delivery can be attributed to the fact that the virus reaches the spinal cord directly leading to efficient transduction (Fig. 3b) and transduces peripheral organs concomitantly (Fig. 4). Consistent with this, the comparison of the SMN levels in the spinal cord of SMNΔ7 mice injected with scAAV9-SMN through ICV and IV demonstrated a greater level of SMN in ICV-injected than IV-injected mice at P7 and P14 [24]. Furthermore, ICV injection of 1 × 1011 genomic copies of scAAV9-SMN extended the life span of the SMNΔ7 mice up to 1 year (Fig. 5a). The SMN levels still remained very high in the spinal cord of the ICV-injected animals at P57 when compared to untreated SMA mice at P7 (Fig. 5b). The ICV-injected SMNΔ7 mice with scAAV9-SMN and untreated SMNΔ7 are photographed at P14 (the end stage of untreated SMNΔ7) which indicates a significant difference in their appearance, body mass, and strength (Fig. 5c).
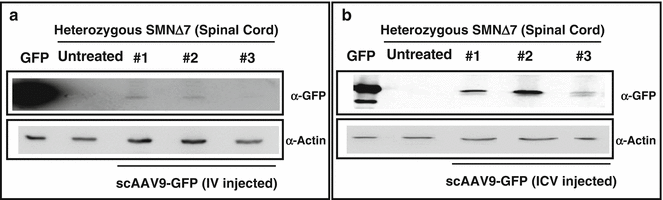
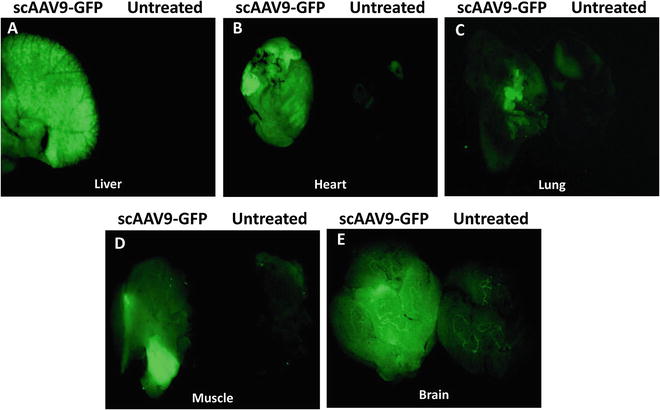
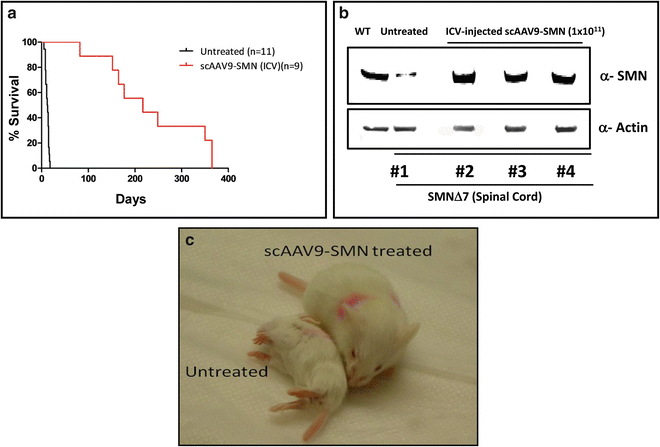
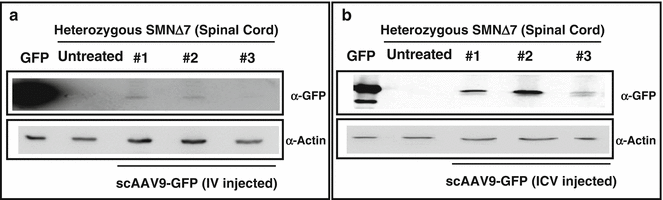
Fig. 3
ICV injection of scAAV9-GFP leads to a higher expression of the GFP in the spinal cord than IV injection. (a and b) Western blot was performed to detect the GFP expression in the spinal cord of the heterozygous (unaffected) SMNΔ7 mice 10 days following IV (a) and ICV (b) injection with 6 × 109 genomic copies of the virus. HeLa cell extracts transfected with the GFP construct were used as a positive control and the spinal cord of the untreated mice as negative control. SMN and actin were detected using monoclonal antibodies
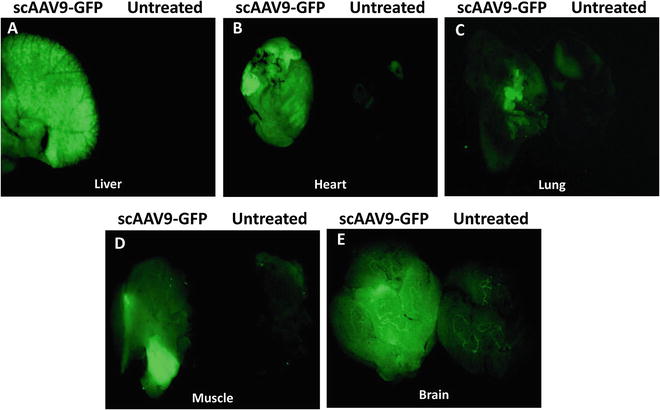
Fig. 4
ICV delivery of scAAV9-GFP at P2 results in the transduction of peripheral organs. Heterozygous SMNΔ7 mice were ICV injected with 1 × 1011 genomic copies of scAAV9-GFP, and the peripheral organs were harvested 5 days postinjection. The organs of virus-injected and age-matched untreated control mice were placed side by side, and the images were captured using a Leica M205 FA Stereomicroscope with the filter excitation of 450–490 nm and emission of 500–550 nm. GFP expression was detected in the liver (a), heart (b), lung (c), muscle (d), and brain (e) of scAAV9-GFP-injected mice
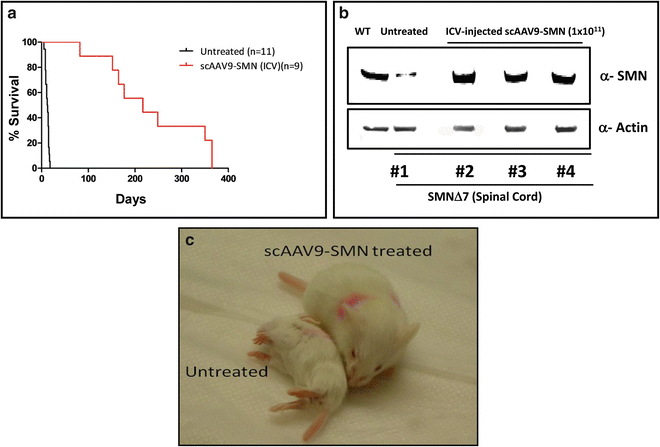
Fig. 5
ICV injection of scAAV9-SMN rescues the SMA phenotype. (a) Kaplan Meier graph indicates percent survival of SMNΔ7 mice injected at P2 with 1 × 1011 genomic copies of scAAV9-SMN through ICV delivery. (b) High levels of SMN protein exist in the spinal cord of SMNΔ7 mice 57 days following ICV delivery of scAAV9-SMN. (c) Photographs of scAAV9-SMN-injected and age-matched untreated SMNΔ7 mice at P14 are shown
1.3 ICV Injection into the Mouse Brain at Early Postnatal Days
ICV injection in vertebrate animals has been utilized in many studies that aimed to investigate the metabolic changes in infectious diseases [88], therapeutic interventions in Alzheimer’s disease [89], SMA [24, 44, 46–48, 59, 80–83], respiratory diseases [90], autism [91, 92], Parkinson’s disease [93], and cardiovascular diseases [94]. Neurodegenerative diseases greatly benefit from therapeutics that can enter directly into the CNS. In humans, this type of delivery is through surgical procedures of intrathecal injection (lumbar puncture) and intraventricular injection. Intrathecal delivery is common in chemotherapy treating leukemia found in the brain and spinal cord and also stem cell therapy for spinal cord injuries [95]. Intraventricular injection in humans is conducted by delivering the therapeutic agent into a specialized catheter called an Ommaya reservoir that is placed into the lateral (outer) ventricle of the brain. This procedure is used most commonly in acute leukemia but can be used in other situations as well. In preclinical studies using mice, CNS delivery can be achieved by injecting directly into the cerebral lateral ventricles (intracerebroventricular) at neonatal stages. This type of injection will result in the delivery of materials into the CNS through the cerebrospinal fluid. The primary benefit is direct delivery into the CNS and effectively bypassing the blood-brain barrier. Therefore, this type of injection will allow the analysis of the viral vector or any other compound that is not fully optimized for drug-like properties (such as blood-brain barrier permeability) for their effectiveness in disease-relevant tissues. Extensive transduction of the CNS by ICV injection is feasible depending on the appropriate choice of viral vector, serotype, viral titer, and more importantly the time of the application. The ICV injections performed by freehand require the skull to be relatively pliable. Therefore, this type of injection is only possible through the first week of life. As the animals age, the hardening of the skull makes this procedure increasingly difficult and harmful to the animals [96].
2 Materials
See Table 1.
Table 1
Materials and tools required for ICV injection into the mouse brain
Materials/tools | Company | Cat. # |
---|---|---|
Food dye | McCormic | N/A |
KimbleTM disposable micro capillary pipettes | Fisher Scientific 13-678-18A | |
P-97 Flaming/Brown micropipette puller | Sutter Instrument N/A | |
Plastic syringe | BD Biosciences | 305269 |
3 Methods
3.1 ICV Injection into the Mouse Brain at Early Postnatal Days
ICV delivery does not require any extensive surgical devices and can be easily performed by a single person. However, the small size of pups at P2–4 and the subsequent small target areas can make the injection challenging and may require a great deal of practice. Our procedure requires a sterilized micropipette glass needle that is attached to a 5 or 10 ml plastic syringe through a long tubing system. A fiber-optic light will be helpful for the visualization of anatomic regions of the brain. A visual demonstration of the ICV injection technique is published previously by our group [96]. Here, we describe a very detailed protocol of the ICV injection with photos demonstrating every step of the process (Figs. 6, 7, 8, and 9).
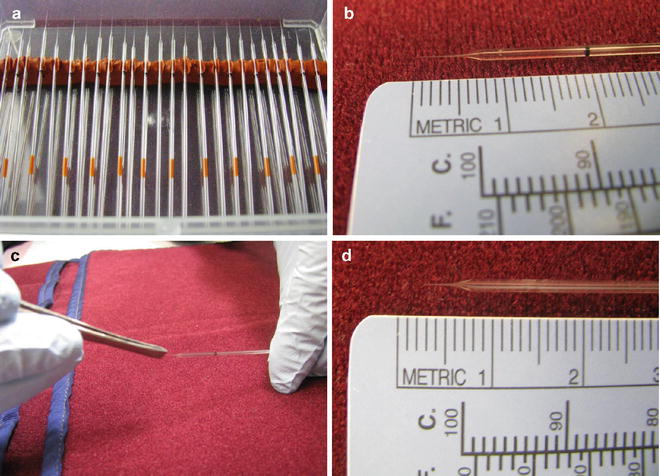
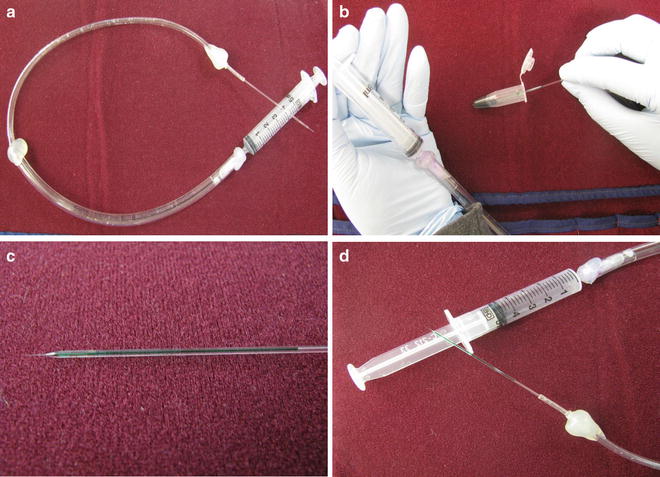
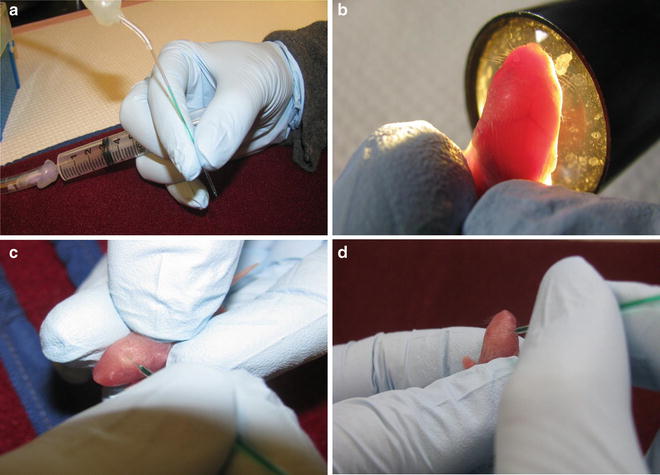
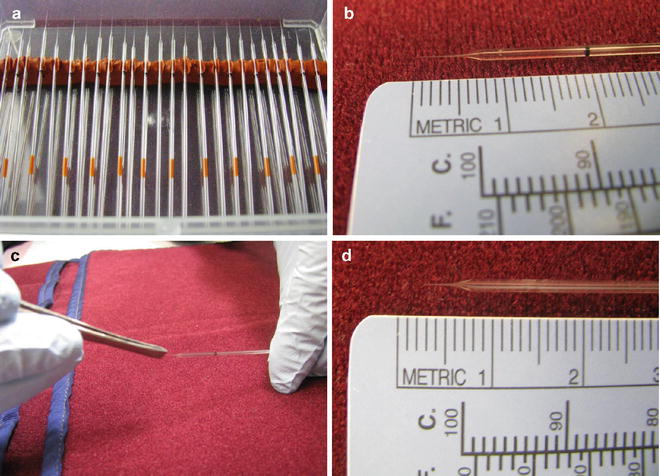
Fig. 6
Proper needles and the proper length of the needle’s tip for ICV injection are illustrated. (a) Number 19 glass micropipette needles. (b) Length of the needle’s tip (~5 mm). (c) Breaking the needle’s tip. (d) Length of the tip, after breaking, for ~2 mm penetration into the mouse cerebral ventricle
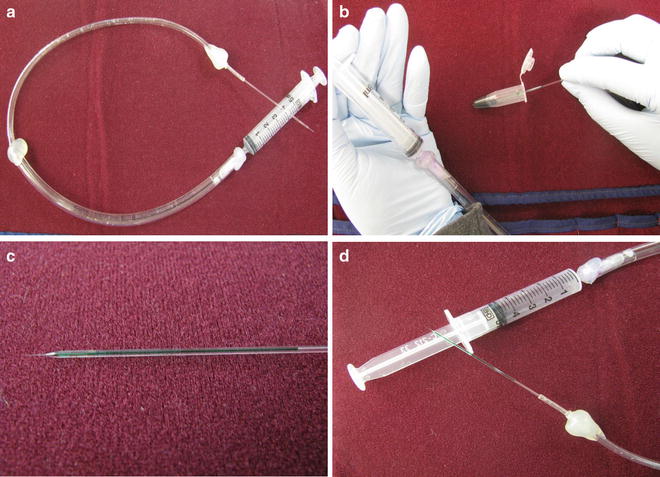
Fig. 7
The steps to upload the injection solution into the needle are illustrated. (a) Needle is attached to the syringe via long tubing. (b) Uptake of the injection solution into the needle by placing the needle inside the injection solution and pulling the plunger of the syringe. (c) Uploaded needle containing approximately 5–7 μl of the injection solution. (d) Uploaded needle is attached to the syringe with pulled back plunger
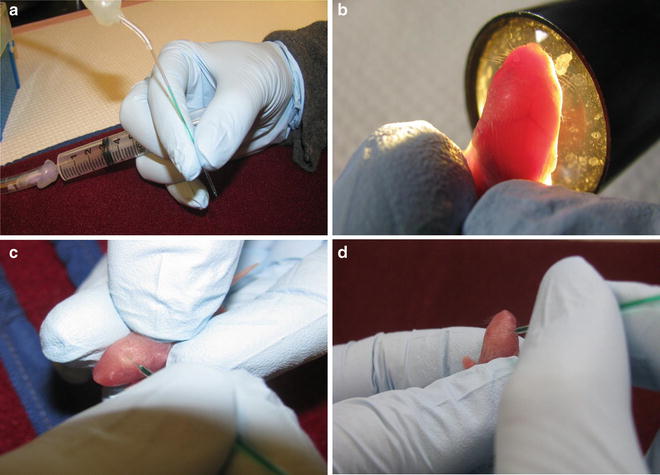
Fig. 8
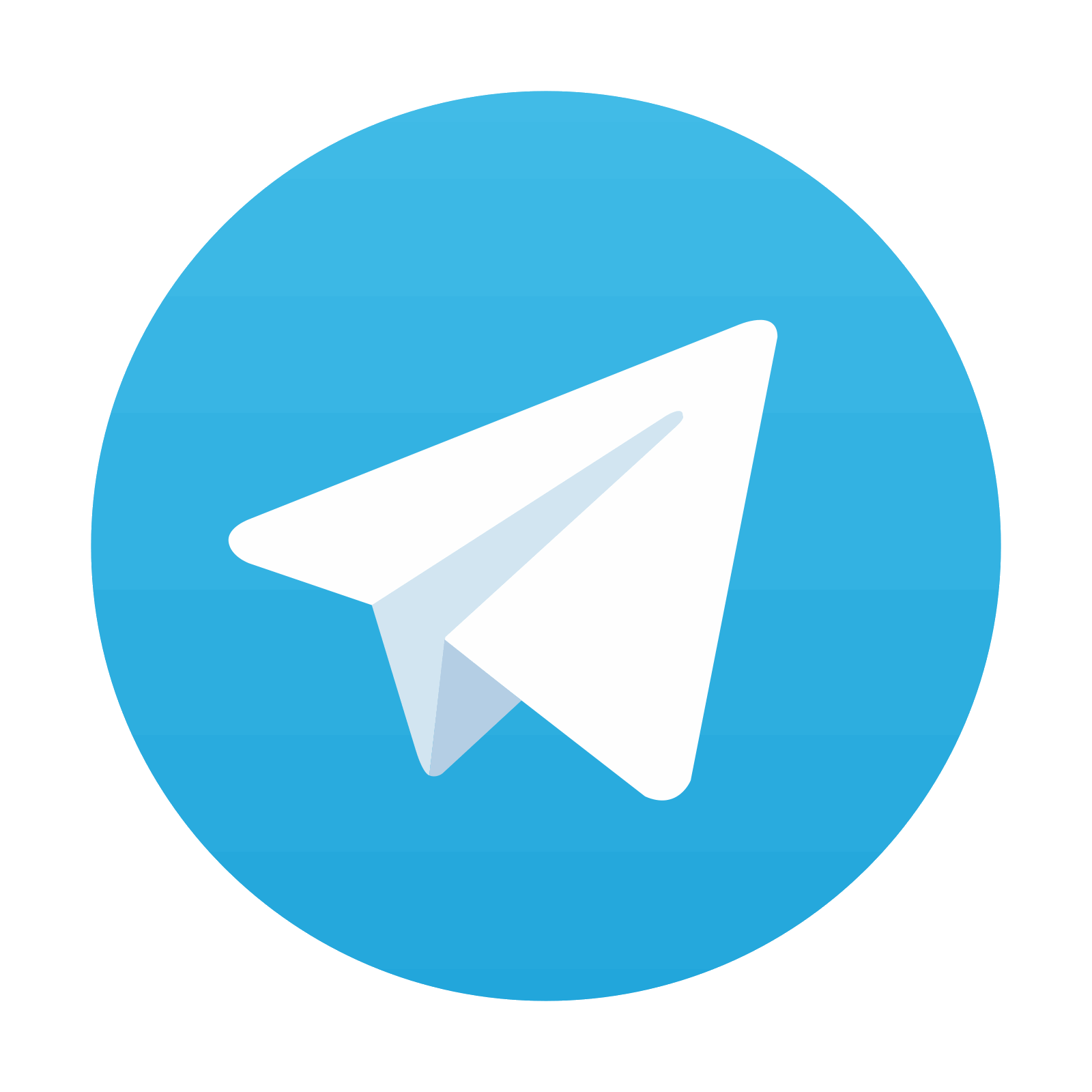
The sequential steps in ICV injection of the mice brain are illustrated. (a) The proper holding of the needle and the syringe during injection. (b) Grasping the pup’s head for the visualization of the anatomical structure of the mouse brain using a fiber-optic light. (c) Penetration of the needle into the left ventricle. (d) Penetration of the needle into the right ventricle
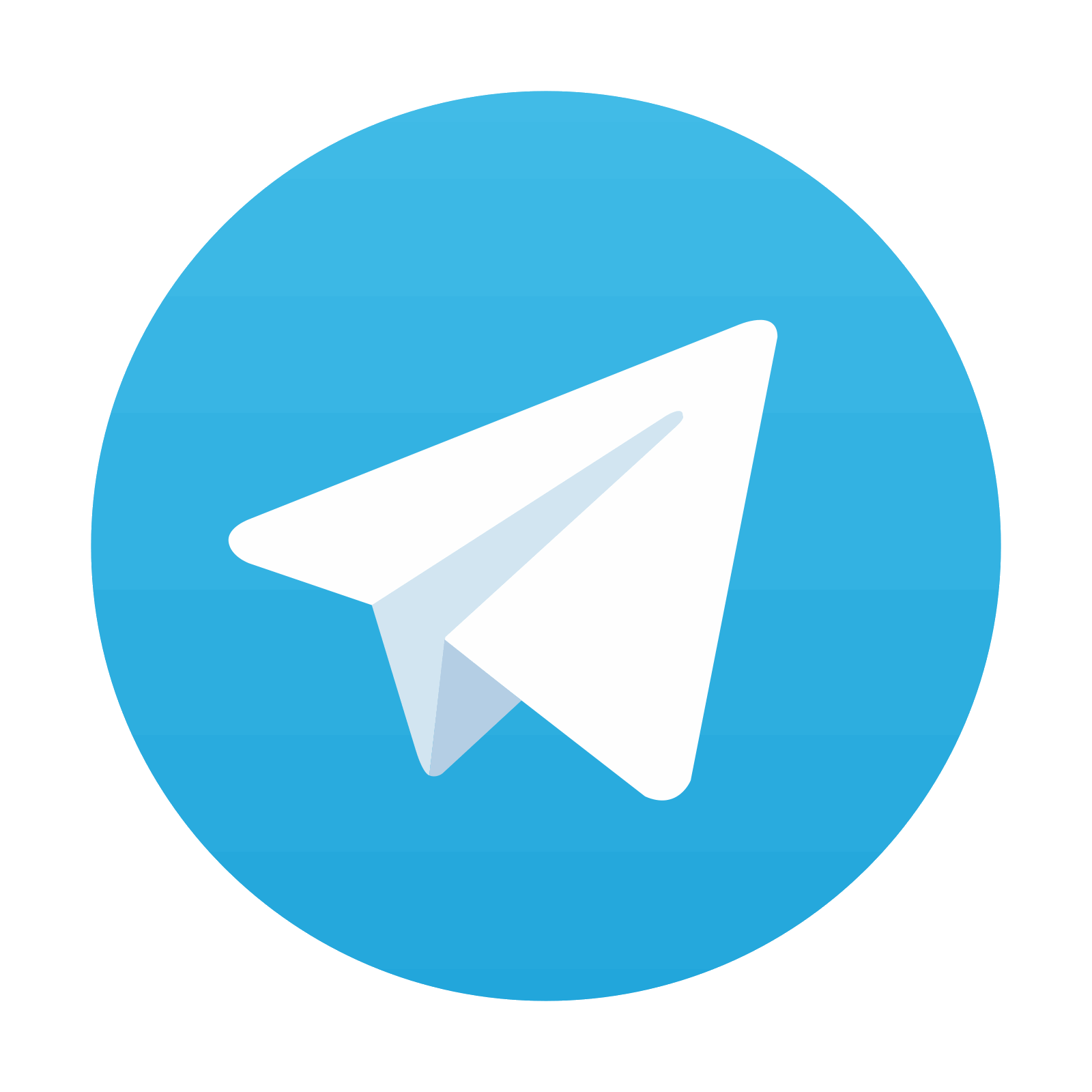
Stay updated, free articles. Join our Telegram channel
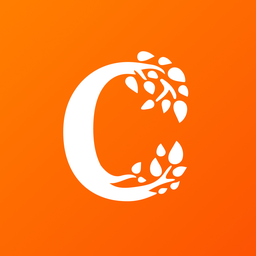
Full access? Get Clinical Tree
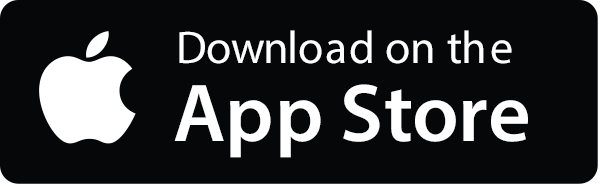
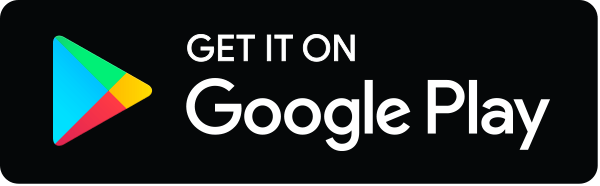
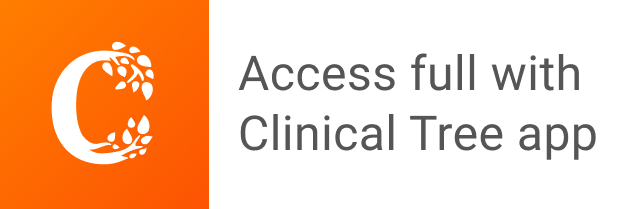