Fig. 4.1
The causes of autism spectrum disorders
In current clinical practice, the diagnosis of ASDs is purely based on the behavioral assessment using various standardized screening or diagnostic tools. There are no reliable biological biomarkers or brain imaging markers to screen or confirm the diagnosis for ASDs. The diagnostic criteria of ASDs related to the behavioral domains have been evolving and are frequently a subject of debate in both professional and public forums. In the Diagnostic and Statistical Manual of Mental Disorders IV (DSM-IV ) (APA 2000), a diagnosis of autism is made based on recognizing impairments in three core domains of social interaction, communication as well as restricted repetitive and stereotyped patterns of behavior, interests, and activities. Furthermore, patients with autism could be diagnosed with four separate disorders: autistic disorder, Asperger’s syndrome, pervasive developmental disorder—not otherwise specified (PDD-NOS) and childhood disintegrative disorder. In the recently published DSM-5 , an umbrella term of autism spectrum disorders is used to cover all separate diagnoses defined in DSM-IV (APA 2013) such that an individual diagnosis such as Asperger’s syndrome will not be used. In DSM-5, the diagnostic criteria of ASDs are based on impairments in two domains (1) persistent deficits in social communication and social interaction across multiple contexts; and (2) restricted, repetitive patterns of behavior, interests, or activities. The severity is based on the level of support that is required to meet the needs of social communication impairments and restricted and repetitive patterns of behaviors. Although this practice has been useful, the limitation is also apparent. Our ability to measure and quantify the impairments of social and communication behaviors in humans is very limited. In many cases with significant comorbidity of intellectual disability, the diagnosis is even more challenging. In DSM-5, the comorbidity of neurological presentations such as intellectual disability and seizures is not considered in the diagnostic criteria of ASDs. Non-behavioral features such as dysmorphic features and congenital anomalies are not taken into account either. However, in the clinical evaluation of ASDs (Schaefer et al. 2013), the probability to uncover a genetic defect is significantly higher in ASD cases with dysmorphic features, congenital anomalies, seizures, and significant intellectual disability (Beaudet 2007; Jacquemont et al. 2006). ASDs are referred to as syndromic if the autistic diagnosis is part of the clinical presentation of a known genetic syndrome or idiopathic if the cause is unknown.
The number of children with the diagnosis of ASDs has been increasing steadily over the last decade. The reported prevalence in children was 1 in 68 in the US in 2014 (DDMNS 2014) compared to 1 in 110 as reported in 2009 (ADDMN 2009) and has a male to female ratio of 4 to 1. The exact underlying reason for the increase in prevalence is not known and is also a subject for debate. The gender difference is also not well understood. There is no clear evidence supporting that mutations in a major gene(s) in the sex chromosomes contribute significantly to the cause in idiopathic ASDs. The alarming increase in the number of new ASD cases has intensified the debate about the role of non-genetic factors, the interaction between genes and the environment , or epigenetics in the etiology of ASDs (Gregory et al. 2013; Hallmayer et al. 2011).
The topic of autism genetics has been reviewed extensively in many recent journal reviews (Devlin and Scherer 2012; Huguet et al. 2013; Miles 2011; Ronemus et al. 2014; Rosti et al. 2014; State and Levitt 2011). In this chapter, we will summarize the key findings from recent ASD genetic and genomic studies using genome-wide copy number variant analysis and next generation sequencing (NGS) techniques, describe the application of these techniques in the clinical genetics evaluation of ASDs, and discuss the challenges of determining the causal role of copy number and sequence variants and genetic counseling dilemmas associated with ASDs in clinics.
4.2 Genetic Basis of ASDs
The considerable clinical heterogeneity observed in ASDs has created a significant challenge to understanding the genetic inheritance underlying the ASDs. The knowledge learned from syndromic ASDs indicated that autistic disorders can be caused by a defect in a single gene. The inheritance of syndromic ASDs becomes clear once the gene implicated in the genetic syndrome is identified. The classic examples in this category are fragile X syndrome and Rett syndrome (Amir et al. 1999; Pieretti et al. 1991), which are also the most common causes for intellectual disability in males and females respectively. With more reports of autism being a clinical feature of different genetic syndromes, the list of syndromic ASDs is growing (Table 4.1). However, because systematic natural history studies have not been conducted for most of these genetic syndromes, these claims may be overstated in some cases or under-appreciated in others. Regardless, knowledge of syndromic ASDs provides a framework for a differential diagnosis in the clinical evaluation of ASDs. The studies of the genes implicated in these syndromic ASDs will also provide insight for the pathophysiology of idiopathic ASD.
Table 4.1
Syndromic ASD
Chr | Gene | Syndromes |
---|---|---|
2 | MBD5 | 2q31 microdeletion sydnrome |
2 | SOS1 | Noonan syndrome |
5 | CDKL5 | Rett-like syndrome |
5 | NSD1 | Sotos syndrome |
7 | CHD7 | CHARGE |
7 | CNTNAP2 | Cortical dysplasia-focal epilepsy syndrome |
7 | RAF1 | Noonan syndrome |
7 | BRAF | Noonan syndrome |
9 | TSC1 | Tuberous sclerosis complex |
12 | CACNA1C | Timothy syndrome |
12 | PTPN11 | PTEN associated disorder |
12 | KRAS | Noonan syndrome |
14 | PTEN | PTEN associated disorders |
14 | FOXG1 | Angelman-like syndrome |
15 | UBE3A | Angelman syndrome |
15 | MAP2K1 | Noonan syndrome |
16 | TSC2 | Tuberous sclerosis complex |
17 | RAI1 | Smith-Magenis syndrome |
18 | TCF4 | Pitt-Hopkins syndrome |
22 | SHANK3 | Related to Phelan-McDermid syndrome |
X | MECP2 | Rett syndrome |
X | FMR1 | Fragile X syndrome |
X | SLC6A8 | Creatine transporter |
X | SLC9A6 | X-linked Angelman-like syndrome |
X | HPRT1 | Lesch-Nyhan syndrome |
X | ARX | ARX related disorders |
X | MED12 | Lujan-Fryns syndrome |
Discussion of the genetic basis in idiopathic ASD cases is more complicated. Mutations in single genes probably account for 5–10 % of ASD cases in all studies in the literature (Buxbaum et al. 2012; Huguet et al. 2013; Schaaf et al. 2011; State and Levitt 2011). Two generation pedigrees with typical ASDs have been rarely reported in the literature. The reduced reproduction fitness in individuals with ASD is often cited as the reason. Although the families with more than one affected child, i.e. multiplex families, are frequently encountered, the clinical presentations among affected siblings usually have more differences than similarities posing an intriguing question as to whether recessive inheritance is a good fit in general. De novo genetic defects including copy number variants and single nucleotide variant s have been the main focus for autism genetic studies conducted over the last decade. As described below, the results from these studies strongly support an important role of de novo and rare genetic mutations in the etiology of ASDs (Devlin and Scherer 2012; Ronemus et al. 2014; State and Levitt 2011).
4.3 Copy Number Variants (CNVs)
Although the term copy number variant is relatively new, the implication of chromosome dosage, i.e. chromosomal deletion and duplication, in genetic diseases have long been recognized for more than two decades. Conceptually, the chromosomal aneuploidy could be considered as the largest CNV in genome that is always pathogenic. Since the discovery of CNVs in the early 2000, the analyses of CNVs have been conducted in a long list of human diseases. The studies of CNVs associated with ASDs and other neurodevelopmental disorders are probably the most productive (Stankiewicz et al. 2003). Autistic behaviors have been described in several well characterized microdeletion and duplication syndromes including Angelman and Prader-Willi , Smith-Magenis, Potoki-Lupski, Velocadiofacial syndrome (VCFS), and Phelan-McDermid syndromes (PMS) (Laje et al. 2010; Phelan 2008; Potocki et al. 2007; Williams et al. 2001). In the case of idiopathic ASD, one of the first and still best examples of an associated chromosomal rearrangement is the maternally inherited duplication of the chromosomal 15q11-q13 Angelman and Prader-Willi syndrome region observed using traditional and high resolution chromosome analysis (Cook et al. 1997). Although a variable phenotype has been reported with this particular duplication, autism, developmental delay, seizures and hypotonia are common features (Ingason et al. 2011; Roberts et al. 2002; Thomas et al. 2006). This rearrangement has been validated in numerous subsequent case reports and large scale genome-wide CNV studies using chromosome microarray analysis (CMA) (Boyar et al. 2001; Bucan et al. 2009; Depienne et al. 2009; Gurrieri et al. 1999; Wang et al. 2004).
In an extensive review of 33 studies including 22,698 patients overall, the International Standard Cytogenomic Array Consortium found that CMA offered a diagnostic yield of 15–20 % as compared to 3 % for G-banded chromosome analysis (Miller et al. 2010) in patients with idiopathic ASDs or intellectual disability (Miller et al. 2010). A set of CNVs highly penetrant for ASDs that were identified through research studies using genome-wide CNV analyses in a large set of ASD cases are listed in Table 4.2 (Bucan et al. 2009; Celestino-Soper et al. 2011; Pinto et al. 2010; Sanders et al. 2011). These studies clearly support an important role of CNVs in the genetic etiology of ASDs.
Table 4.2
Clinically relevant ASD associated CNVs
Locus | Candidate gene | Other associated disorders |
---|---|---|
1q21.1 | ND | ID/SCZ/BD |
2p16.3 | NRXN1 | ID/SCZ |
2q23.1 | MBD5 | ID/SE |
3q29 | DLG1/PAK2 | ID/SCZ |
7q11.23 | LIMK | ID |
11q13.3 | SHNAK2 | ID/SE |
15q11.2 | UBE3A | ID/SE |
15q13.3 | CHRNA7 | ID/SCZ |
16p11.2 | KCD13? | ID/BD/SCZ |
22q11.2 | ND | ID/SCZ |
22q13.3 | SHANK3 | ID/SE/BD |
Xp22.1 | PTCHD1 | ID/SE |
Xp22.3 | NLGN3 | ID |
Xq13.1 | NLGN4X | ID |
It has been suggested that rare CNVs containing genes involved in neural plasticity or synapse formation and maintenance as well as chromatin modification are associated with ASDs and intellectual disability and that disruption of these shared biological pathways can result in neurodevelopmental disorders (Huguet et al. 2013; Pinto et al. 2014; Toro et al. 2010). In a study comparing 996 ASD individuals of European ancestry to 1287 matched controls, ASD cases were found to carry a higher global burden of rare CNVs, especially for loci previously implicated in either ASD and/or intellectual disability (Pinto et al. 2010). Pinto et al. (2010) analyzed 2446 ASD-affected families and confirmed an excess of gene deletions and duplications in affected versus control groups (Pinto et al. 2014). Genes affected by de novo CNVs and/or loss-of-function single-nucleotide variants converged on networks related to neuronal signaling and development, synapse function, and chromatin regulation. Among the CNVs identified, there were numerous de novo and inherited events, sometimes in combination in a given family, implicating many novel ASD genes including CHD2, HDAC4, GDI1, SETD5, MIR137,and HDAC9.
Recently, an exon-targeted oligo array was used to detect intragenic copy number variants in a cohort of 10,362 patients including those with a clinical indication of ASDs (Boone et al. 2010). The more commonly observed CNVs detected in this cohort include those affecting known and candidate genes for ASDs such as NRXN1, CNTNAP2, NLGN4X, A2BP1(RBOX1), CNTN4, CDH18, and TMEM195. A more detailed clinical and molecular characterization of 24 patients who have intragenic deletions of NRXN1 revealed that the seventeen patients with deletions involving exons manifested developmental delay/intellectual disability (93 %), infantile hypotonia (59 %) and ASDs (56 %) (Schaaf et al. 2012). These results indicate that a small intragenic deletion is significant enough to contribute to neurodevelopmental disorders, including ASDs, but the detection of these defects could be easily missed by array designs that lack exonic coverage.
Detection of recurring intragenic CNVs in this patient population will support reclassifying candidate genes implicated in neurodevelopmental disorders as disease-causing genes. For example, two patients with developmental delay and ASDs were identified with small duplication CNVs involving single exons that disrupt AUTS2 (Nagamani et al. 2013). This finding was further verified by another study comparing 17 well-characterized individuals with microdeletions affecting at least one exon of AUTS2 which enabled the identification of a variable syndromic phenotype including intellectual disability (ID), autism, short stature, microcephaly, cerebral palsy, and facial dysmorphisms (Beunders et al. 2013). These results illustrate the different sensitivities for detecting genetic defects based on the resolution of different arrays. However, comparisons among different array designs to detect disease-causing defects in a clinical setting have not been fully evaluated.
The results from research studies and clinical tests not only have confirmed the previous observations from individual case reports but also consolidated several major findings related to the role of CNVs in ASDs as follows: (1) A number of new CNVs are strongly implicated in ASDs but also show both variable expressivity and pleiotropic effects; (2) between 5–10 % of previous idiopathic ASD cases will carry rare CNVs; (3) both de novo and inherited CNVs confer a risk in ASD; and (4) there is a 3 fold increase in large and rare de novo CNVs in probands compared to their siblings (Buxbaum et al. 2012; Devlin and Scherer 2012). These findings, in general, support the clinical application of copy number analysis in ASDs as the recommended first tier molecular test in the clinical genetics evaluation of ASDs (Miller et al. 2010; Schaefer et al. 2013). However, the translation of these findings into clinical practice remains a challenge because the causal role of these CNVs cannot always be firmly established as discussed below.
4.4 Single Nucleotide Variants (SNVs)
The identification of genes implicated in syndromic ASDs indicated that a sequence change of a single nucleotide in a single gene can confer a significant genetic risk for ASDs. This principle has been validated in idiopathic ASDs using a candidate gene sequencing approach prior to the emergence of next generation sequencing . One of the best examples is the identification of mutations in the SHANK3 gene (Durand et al. 2007). SHANK3 was mapped to the critical region of chromosome 22q13.3 in Phelan-McDermid syndrome (PMS) in which autism is a prominent feature (Bonaglia et al. 2006; Durand et al. 2007; Wilson et al. 2003). Subsequently, pathogenic point mutations in SHANK3 have been identified in idiopathic ASD in many independent reports (Boccuto et al. 2013; Moessner et al. 2007). Similar scenarios have also contributed to the discovery of other genes such as MBD5, CNTNAP2, Neuroligins and Neurexins , all of which are implicated in ASDs (Arking et al. 2008; Laumonnier et al. 2004; Talkowski et al. 2011; Zweier et al. 2009). The low frequency of these sequence variants posed a challenge in experimental design for discovering new candidate genes until the recent emergence of the new generation sequencing (NGS) technique. Whole exome (WES) and whole genome sequencing (WGS) by NGS have provided an unprecedented opportunity to discover rare variants throughout the genome in ASDs. To date, there are multiple large scale ASD WES and several small scale WGS studies that have been carried out in trios, namely the proband plus the unaffected biological parents (Bi et al. 2012; Chahrour et al. 2012; Iossifov et al. 2012; Jiang et al. 2013; Michaelson et al. 2012; Neale et al. 2012; O’Roak et al. 2012; Sanders et al. 2012; Shi et al. 2013). The majority of these studies were focused on the analysis of de novo mutations. Several studies analyzed both rare inherited and de novo mutations (Chahrour et al. 2012; Lim et al. 2013; Yu et al. 2013). Among more than 1000 families assessed by these studies, the presence of de novo loss-of-function (LOF) mutations was consistently significantly higher in probands compared to controls. These studies have led to confirmation of many known genes and also the discovery of more than a dozen new genes with a high confidence of a causal role in ASDs (Table 4.3). A large number of de novo missense variants (10 folder high than LOF allele) have also been identified in ASDs from WES studies. Some of these missense changes are certain to contribute to the risk of ASD susceptibility. However, only 5–10 % excess of such mutations was found in ASD cohorts compared to controls, which did not reach statistical significance collectively. It is not possible to assign risk for these missense variants confidently without functional studies or through validation in an even larger number of cases. Only three ASD WGS studies have been reported so far (Arking et al. 2008; Chahrour et al. 2012; Sanders et al. 2012). In contrast to WES, the sample sizes of those evaluated by WGS are relatively small. In one study, WGS of 32 trios detected clinical relevant variants in 16 probands (Jiang et al. 2013). This study supports the feasibility of using WGS as a clinical test and validated the improved sensitivity of WGS compared to WES in detecting pathogenic sequence variants. In addition, the WGS has detected an average of 50–70 de novo sequence variants in non-coding regions. It is even more challenging to determine the clinical relevance of these findings to ASDs.
Table 4.3
ASD candidate genes from WES/WGS studies
Chr | Gene symbol | Description | MUTATIONS |
---|---|---|---|
1 | POMGNT1 | Protein O-linked mannose beta1,2-N-acetylglucosaminyltransferase | p.R367H |
1 | NTNG1 | Netrin G1 | p.T135I (58); p.Y23C |
1 | POGZ | Pogo transposable element with ZNF domain | Frame shift (57); Frame shift |
1 | USH2A | Usher syndrome 2A (autosomal recessive, mild) | p.W2075X, p.Y4238X, compound heterozygous |
2 | DNMT3A | DNA (cytosine-5-)-methyltransferase 3 alpha | p.R635W |
2 | ARID5A | AT rich interactive domain 5A (MRF1-like) | p.G120V |
2 | IFIH1 | Interferon induced with helicase C domain 1 | Splicing site |
2 | SCN2A | Sodium channel, voltage-gated, type II, alpha subunit | p.G1013X; p.C959X; deletion |
2 | SCN1A | Sodium channel, voltage-gated, type I, alpha subunit | p.P1894 L (1 missense in additional cases) |
3 | AMT | Aminomethyltransferase | p.I308F; p.D198G |
5 | GPR98 | G protein-coupled Receptor 98 | p.D6252N (3 point mutations in additional cases) |
6 | PEX7 | Peroxisomal biogenesis factor 7 | p.W75C |
6 | SYNE1 | Spectrin repeat containing, nuclear envelope 1 | p.L3206M |
6 | VIP | Vasoactive intestinal peptide | p.Y73X (60) |
8 | VPS13B | Vacuolar protein sorting 13 homolog B (yeast) | Frame shift; p.S824A |
8 | PKHD1L1 | Polycystic kidney and hepatic disease 1 (autosomal recessive)-like 1 | Splicing site |
9 | LAMC3 | Laminin, gamma 3 | p.D339G (1 missense in additional cases) |
10 | ANK3 | Ankyrin 3, node of Ranvier (ankyrin G) | p.G3690R |
10 | USP54 | Ubiquitin specific peptidase 54 | Frame shift |
11 | MICALCL | MICAL C-terminal like | Frame shift |
11 | CAPRIN1 | Cell cycle associated protein 1 | p.Q399X |
11 | KIRREL3 | Kin of IRRE like 3 (Drosophila) | Downstream (3 point mutations in additional cases) |
12 | GRIN2B | Glutamate receptor, ionotropic, N-methyl D-aspartate 2B | Splicing site (1 nonsense and 1 frame shift in additional cases) |
12 | NCKAP5L | NCK-associated protein 5-like | p.G11D |
12 | PAH | Phenylalanine hydroxylase | Deletion; p.Q235X |
12 | UBE3B | Ubiquitin protein ligase E3B | p.R40C |
14 | CHD8 | Chromodomain helicase DNA binding protein 8 | 3 Lof mutations in additional cases; p.Q959X; Frame shift |
14 | KIAA0284 | Centrosomal protein 170B | p.R1122H |
16 | ABCC12 | ATP-binding cassette, sub-family C (CFTR/MRP), member 12 | p.W1024X |
17 | ZNF18 | Zinc finger protein 18 | p.H377N |
18 | KATNAL2 | Katanin p60 subunit A-like 2 | Splicing site; 3 Lof mutations in additional cases |
20 | KCNQ2 | Potassium voltage-gated channel, KQT-like subfamily, member 2 | Frame shift |
21 | DYRK1A | Dual-specificity tyrosine-(Y)-phosphorylation regulated kinase 1A | Splicing site; Frame shift |
22 | CLTCL1 | Clathrin, heavy chain-like 1 | p.R125C |
X | NLGN4X | Neuroligin 4, X-linked | p.Q329X |
X | KAL1 | Kallmann syndrome 1 sequence | p.R423X |
X | WWC3 | WWC family member 3 | p.R940Q |
X | ZC3H12B | Zinc finger CCCH-type containing 12B | p.R318Q |
X | DGAT2L6 | Diacylglycerol O-acyltransferase 2-like 6 | p.R151X; p.Q214X |
X | KIAA2022 | KIAA2022 | p.Q1471X |
X | PCDH11X | Protocadherin 11 X-linked | Splicing site |
X | SRPX2 | Sushi-repeat containing protein, X-linked 2 | Splicing site |
X | LUZP4 | Leucine zipper protein 4 | p.R161X; p.R163X |
X | BCORL1 | BCL6 corepressor-like 1 | p.R1090P |
X | AFF2 | AF4/FMR2 family, member 2 | p.A283X |
X | MECP2 | Methyl CpG binding protein 2 | p.E495X; p.E483X |
X | TMLHE | Trimethyllysine hydroxylase, epsilon | Splicing site |
In contrast to the convincing evidence supporting the pathogenicity of rare CNVs and SNVs in ASDs, the implication of common variants remains tenuous (Devlin et al. 2011; Devlin and Scherer 2012). Several large and independent genome-wide association studies have been conducted to identify common variants that exert significant risk for ASDs (Anney et al. 2010; Szatmari et al. 2007; Wang et al. 2009; Weiss 2009). Two earlier large studies (> 3000 subjects) reported a significant association of two different loci at 5p14.1 and 5p15.2, respectively (Wang et al. 2009; Weiss et al. 2009). However, these loci have not been replicated in other studies including WES analysis. Although further investigation may be necessary to clarify the reason underlying the differences among the studies, it is probably fair to conclude that common variants may not have a significant impact on ASDs (Devlin et al. 2011; Zecavati and Spence 2009).
4.5 Biochemical Defects and Mitochondrial Dysfunction
Numerous metabolic abnormalities have been reported in the context of an ASD phenotype but these are usually not specific for a certain diagnosis (Schaefer et al. 2013; Zecavati and Spence 2009). Individual metabolic disorders associated with an ASD phenotype are relatively rare (Table 4.4). However, all together, these disorders may contribute to ~ 3 % of all ASD causes evaluated in clinical genetic clinic. For example, severe ASD is well recognized in individuals with untreated or partially treated phenylketonuria (PKU). Several studies have suggested a link between mitochondrial dysfunction and ASDs (Giulivi et al. 2010; Guevara-Campos et al. 2013; Legido et al. 2013; Rossignol and Frye 2012). However, it remains to be determined whether the mitochondrial dysfunction is primary or secondary to an unidentified defect. Most metabolic disorders in a classical form including mitochondrial diseases are associated with other clinical symptomatology such as seizures and extrapyramidal signs as well as biochemical disturbances. However, it is not known whether the atypical or mild presentations of metabolic disorders may present more commonly as an ASD phenotype and are under-detected.
Table 4.4
Metabolic diseases with ASD phenotype
1. | Untreated PKU |
2. | MTHFR |
3. | 3β-Hydroxycholesterol-7-reductase deficiency |
4. | 6-N-trimethyllysine dioxygenase deficiency |
5. | Adenylosuccinate lyase deficiency |
6. | Cerebral folate deficiency |
7. | Disorders of creatine transporter |
8. | Mitochondrial Diseases? |
4.6 Diagnostic Techniques for ASDs in Genetics Clinic
The current practice in the US is that a clinical genetics evaluation is recommended for all children with a confirmed diagnosis of ASD (Johnson and Myers 2007; Schaefer et al. 2013; Zwaigenbaum et al. 2009). It is estimated that a specific genetic etiology can be determined in about 15–20 % of individuals with an ASD (Schaefer et al. 2013). However, high quality or unbiased clinical data to validate the real yield of a clinical genetics evaluation in ASD is lacking (Herman et al. 2007; Shen et al. 2010). The variable practice models among clinics and different diagnostic platforms used in molecular diagnostic laboratories complicates the assessment of validity. A flowchart for the proposed clinical genetics evaluation of ASDs is presented in Fig. 4.2, which highlights the incorporation of new testing methodologies for determining the molecular defect.
4.7 Copy Number Variant Analysis
Detection of copy number variants is usually achieved through array technology and is now the recommended first tier genetic test for the evaluation of ASDs (Miller et al. 2010; Schaefer et al. 2013). Initially, CMA via array comparative hybridization (aCGH) was used for CNV analysis. Single nucleotide polymorphim (SNP) arrays were then introduced and enabled detection of copy number neutral regions of absence of heterozygosity (AOH) in addition to copy number analysis. The level of genomic resolution achieved by an array analysis depends on the number, size and distance between the interrogating probes. Array CGH utilizes oligonucleotides (60–85 mers) while SNPs (25–50 mers) are the probes for SNP arrays, of which millions of both probe types can be synthesized on one glass slide (Ou et al. 2008). There are also customized arrays that can detect single exon CNVs involving only a few hundred base pairs (Boone et al. 2010). However, because SNPs predominate in non-coding regions, it is possible that certain regions of the genome such as single exons may not be represented on SNP arrays. In addition, SNP arrays demonstrate a lower signal-to-noise ratio per probe than CMA, which can result in less sensitivity for detecting CNVs. Therefore, to maximize clinical sensitivity, platforms that are a combination of aCGH and SNP genotyping are becoming the predominant type of array used clinically (Papenhausen et al. 2011; Peiffer et al. 2006; Schwartz 2011; Wiszniewska et al. 2014).
An advantage of SNP arrays is that they can reveal regions within the genome that lack heterozygosity, i.e. absence of heterozygosity. Identification of these regions is useful clinically in detecting polyploidy, uniparental disomy, consanguinity and recessive diseases although these are thought to be infrequent causes of ASDs (Alkan et al. 2011). However, a recent study reported that ASD individuals with intellectual disability have more regions of AOH compared to their unaffected siblings (Gamsiz et al. 2013). Furthermore, analysis of the specific ASD AOH regions aided in the discovery of autism candidate genes by either identifying single genes within an AOH interval that are recurrent in ASD or harbor a homozygous, rare deleterious variant upon analysis of exome-sequencing data (Gamsiz et al. 2013).
While the CNVs listed in Table 4.2 represent large-scale, recurrent CNVs that were detected from the first generation of clinical arrays, the increased resolution of arrays currently being used has promise for discovering more ASD-associated CNVs or individual candidate genes. For example, the combined analyses of CNVs in exonic regions and sequencing of coding exons led to a diagnosis of an autosomal recessive disorder by unmasking a recessive allele in a patient with autism. In this case, a deletion of exons 22–25 in the VPS13B gene, which is associated with Cohen syndrome, was first detected by CNV analysis using an exon-targeted array (BCM V8) and subsequent sequence analysis of the VPS13B gene identified a missense mutation in the other allele (Cohen et al. 2005; Wiszniewska et al. 2014).
These examples highlight that, to discover the precise molecular etiology , it may be necessary to combine different technologies within one test (array CGH + SNP) or use a combination of molecular diagnostic tests (CNV analysis plus medical re-sequencing of candidate genes or whole exome sequencing ).
4.8 Single Nucleotide Variant Analysis
4.8.1 Single Gene Test for Syndromic ASDs
If the patient’s clinical presentation strongly suggests a syndromic ASD, the most effective approach is to request the specific gene test for the suspected genetic syndrome. A list of single genes associated with syndromic ASDs is found in Table 4.1. There are numerous molecular diagnostic tests utilized for single gene testing depending on the type of causative mutation usually observed for that specific gene. Testing methodologies include sequencing analysis (point mutations), FISH (microdeletions/duplications), Southern blotting (large repeat expansions) and multiplex ligation-dependent probe amplification (MLPA, small deletions/duplications). According to the practice guidelines of the American College of Medical Genetics (ACMG) and American Academy of Pediatrics (AAP), DNA testing for fragile X is recommended for all children suspected for an ASD (Myers and Johnson 2007; Schaefer et al. 2013). With the application of CMA, the value of FISH analysis has been phased out gradually in clinical diagnostic practice. However, for a defined microdeletion syndrome with characteristic clinical features, FISH still has its value because of its relative low cost compared to CMA.
4.8.2 Targeted Gene Panels
The development of targeted gene panels (TGP) using NGS technology has been a popular approach in molecular diagnosis practice (Rehm et al. 2013). TGP allows a greater depth of coverage compared to a single gene test and better analytical sensitivity and specificity compared to WES described below. The basic design is to group the genes that are implicated in a disorder with significant genetic heterogeneity or are relevant to particular clinical phenotypes that share the same molecular pathophysiology. TGP is particularly attractive for a disorder with extensive locus heterogeneity that is difficult to differentiate based on clinical presentations. Representative examples include but are not limited to retinitis pigmentosa, Bardet-Bidel syndrome, hearing loss and Noonan syndrome.
In the case of ASDs, the extensive molecular heterogeneity is well recognized despite the fact that the molecular basis for the majority of cases is still not known. Therefore, TGP is an attractive diagnostic strategy for ASDs. Several ASD-related gene panels are offered by clinical molecular diagnostic laboratories and include genes implicated in syndromic ASDs or candidate genes from recent medical re-sequencing studies. The results from WES and WGS have not yet been incorporated in these diagnostic panels. The clinical validity of these panels has not been fully evaluated but it is expected that they will be a useful tool in the clinical setting.
The development of an updated version of an ASD TGP that incorporates the ASD candidate genes from the recent WES and WGS studies is expected to be the next logical step. Although many candidate genes suggested from WES or WGS research studies remain to be validated, it is probably reasonable to include them in the updated version of ASD TGP. This design is a good transition strategy until the use of WES or WGS techniques described below are fully validated for ASDs. The findings from the use of clinical TGP may speed the validation of research findings if the clinical laboratories share results and clinical phenotypes.
4.8.3 Whole Exome Sequencing (WES)
The application of WES in clinical genetics practice has gained momentum recently (Gahl et al. 2012; Jacob 2013; Yang et al. 2013). The proof of principle study of using WES in clinical application was first published in 2009 (Ng et al. 2009). In this study, the success of identifying a disease-causing mutation in a patient with a known genetic syndrome was demonstrated by WES in a research protocol. Subsequently, the success of using WES to discover new disease-causing genes was reported in 2010 (Gahl et al. 2012; Gilissen et al. 2011; Hoischen et al. 2011). In 2011, clinical molecular diagnostic laboratories started to offer WES as a clinical test using different array capture platforms and analytic paradigms (Fig. 4.3). Currently, multiple academic and commercial laboratories in US are offering the clinical WES. The indication for WES as a clinical test is quite variable among clinical geneticists or physicians in other specialties. Patients with significant intellectual disability, seizure disorders, multiple congenital anomalies and other unusual clinical presentations are typically good candidates for WES. Because a significant sub-set of individuals with ASDs, particularly the severe end of ASDs, present with comorbidity of severe intellectual disability, seizure disorders, and other minor congenital anomalies, it is quite reasonable to argue that clinical WES is indicated in these cases if first tier testing is negative (Fig. 4.2). The exact sensitivity of WES in a clinical application cannot be easily obtained. However, accumulated evidence in one clinical laboratory in the US reported an approximately 25 % success rate in determining the cause for ~ 250 cases with variable clinical presentations referred for WES (Yang et al. 2013). Another group in the Middle East reported the finding of 37 potential variants in 100 probands for known Mendelian diseases (37 %) (Rodriguez-Flores et al. 2014). The overall positive detection rate in ASD research WES studies is less than 5 %. However, the results from recent ASD WES/WGS research studies should not be used to estimate the positive predictive value for WES in the clinical setting because the study subjects were not carefully selected and the focus was to identify de novo mutations.
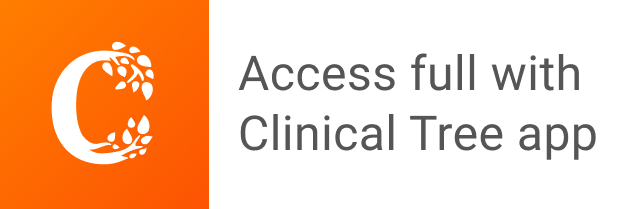