4 E. W. Johnson and Joseph M. Zabramski Cerebral cavernous malformations (CCMs) are vascular anomalies of the central nervous system (CNS) that affect 0.4 to 0.5% of the population.1,2 CCMs occur in two distinct forms: a sporadic form and a familial, or inherited, form. Patients with the sporadic form usually have a single isolated lesion and no family history of neurologic illness. In contrast, the familial form is characterized by multiple lesions and a strong family history of seizures.3 Indeed, the presence of multiple CCMs on magnetic resonance imaging (MRI) studies is almost pathognomonic for the familial form of this disease.4 In the recent MRI study of 132 confirmed carriers of the CCM1/KRIT1 mutation, Denier and coworkers5 reported that 80% of patients had multiple lesions on T2-weighted MR images and 90% had multiple lesions on gradient-echo images. The mean number of lesions per subject was 4.9 ± 7.2 on T2-weighted images and 19.9 ± 33.2 on gradient-echo sequences. Even in this highly selective population, 10% of confirmed CCM1 carriers had only one lesion on gradient-echo MR images. Eight patients (all asymptomatic carriers) had no lesions identified on T2-weighted images, emphasizing the importance of gradient-echo imaging and a careful family history in diagnosing the familial nature of CCMs in some patients. The first suggestion that CCMs might be a hereditary disease appeared in the German medical literature in 1928.6 Sporadic reports of the familial occurrence of CCMs followed, but studies were hampered by the lack of a reliable screening tool to identify affected individuals.7–9 In 1982, Hayman and colleagues convincingly demonstrated that the familial form of CCM was inherited as an autosomal dominant disorder.10 Their report emphasized the variable clinical penetrance of this disorder as well as the limitations of computed tomography (CT) for the detection of these lesions. By the mid-1980s, the MRI characteristics of CCMs were well established, for the first time making it possible to screen patients accurately for this disease.11–15 In 1988, Mason and coworkers16 and Rigamonti and colleagues3 published reports confirming the autosomal dominant inheritance of CCMs in Hispanic families. In 1994, Kurth et al.17 used linkage analysis on genetic material collected from patients in the latter study to map the affected gene, designated CCM1, to the long arm of chromosome 7. Subsequent work by this group and others rapidly confirmed linkage of CCM1 to the same region of 7q and identified a founder effect in Hispanics of Mexican-American heritage: More than 70% of documented CCMs and almost all familial CCMs in this group can be attributed to mutations at CCM1 from one common ancestor.15,18–22 Linkage to CCM1 was also identified in non-Hispanic families. Within these studies, however, were reports of many families with either weak or no clear linkage to the CCM1 locus. In 1998, Craig and coworkers23 reported linkage to two additional loci: CCM2 on the short arm of chromosome 7 (7p13–15) and CCM3 on the long arm of chromosome 3 (3q25.2–27). Within the general population, 40% of families with familial CCM link to CCM1, 20% link to CCM2, and 40% link to CCM3 or to other yet unidentified CCM loci.23,24 This chapter reviews the available information on these loci, including the gene products and their potential role in the pathophysiology of CCM. In 1994, collaborators at the Center for Medical Genetics in Marshfield, Wisconsin, and at the Barrow Neurological Institute in Phoenix, Arizona, linked the first gene for familial CCM, designated CCM1, to chromosome 7q in a large Hispanic family.17,22 Subsequent work by these investigators and others led to identification of the CCM1 gene at 7q21 as Krev-1 interaction trapped 1 (KRIT1).25,26 More than 100 mutations have been identified at the KRIT1 locus.24–39 All mutations identified to date are putative, loss-of-function mutations, including frameshifts, nonsense, and invariant splice site mutations. As its name implies, KRIT1 was initially identified because of an interaction with Krev-1/Rap1a, a member of the Ras family of guanosine triphosphatase (GTPases) with tumor-suppressor activity for Ras oncogenes.40 Thus, initial hypotheses for mechanisms of CCM1 pathogenesis focused on KRIT1 as a regulator of Rap1a-dependent cell proliferation. Extending this theme, Rap1a functions as a Ras antagonist. Loss of KRIT1 in CCM1 patients was hypothesized to perturb this molecular relationship.41 In this model, KRIT1 acts as a tumor-suppressor gene. In support of this hypothesis, the Krev-1/Rap1a pathway is known to be altered in type 2 tuberous sclerosis (TSC2), an autosomal dominant condition characterized by benign neurocutaneous tumors.42,43 The TSC2 gene product, tuberin, functions as a tumor-suppressor protein for Krev-1/Rap1a. Somatic inactivation or loss of heterozygosity of the wild-type tuberin allele in TSC2-associated tumors leads to unregulated growth.43,44 A two-hit model, with a central tumor-suppressor function for KRIT1 in CCM1, would be similar to the mechanism of tuberin inactivation in TSC2. In this model, mutations in CCM1 would lead to unregulated endothelial proliferation. Although a possible mechanism, the dilated capillaries observed pathologically in these lesions appear to reflect a defect in organization or adhesion more than in proliferation. Since the initial identification of KRIT1 by its association with Rap1a, the KRIT1 sequence has been verified to encode for an additional 207 amino acids at the 5′ end of the cDNA.37,45 Importantly, this additional segment has been shown to harbor multiple CCM1 mutations.24,33,36,37,46 Two groups have subsequently identified integrin cytoplasmic domain-associated protein α (ICAP-1α) as a KRIT1-binding partner for this extended protein.47,48 ICAP-1 α is a modulator of β1 integrin signal transduction, a means whereby mammalian cells sense and respond to the extracellular matrix as well as to other cells. In a vascular context, integrins are crucial in the regulation of cell adhesion and migration during angiogenesis.49 Antibodies to β1 integrin block the formation of capillaries, and β1 null cells fail to form blood vessels when tumors are implanted in mice.50–52 Maturation of blood vessels in the CNS is accompanied by marked upregulation of β1 integrin expression, suggesting that integrins are also important in maintaining endothelial function in the adult CNS.53 Using in situ hybridization, Denier et al.54 have demonstrated that KRIT1 is expressed preferentially in neural and epithelial tissues in both the embryonic and adult mouse. Immunohistochemical staining with antibodies to KRIT1 protein demonstrated a similar distribution in human tissues.55 KRIT1 localizes to the vascular endothelium of capillaries and arterioles in a broad variety of human organs. In the brain, expression was identified in both the endothelial cells of the capillaries and in the astrocytic foot processes that make up the blood-brain barrier. These findings suggest that perturbation of integrin-mediated cell adhesion via the KRIT1/ICAP-1α pathway may result in impaired formation or maintenance of capillaries. To explore the role of CCM1 in development, Whitehead and colleagues56 generated a CCM1 mutant allele in the mouse. Heterozygous mutant mice (CCM1+/-) were phenotypically normal and indistinguishable from wildtype embryos; however, homozygous mutant embryos (CCM1-/-) suffered from generalized developmental arrest in midgestation and were disintegrating by E10. Defective development in CCM1-/- embryos was first observed in the vascular system in the vessels of the cephalic mesenchyme. These vessels are destined to invade the neural tube and begin formation of the intracranial vasculature at E10.0, a stage of development that the homozygous mutant embryos fail to reach. The precursor vessels become progressively dilated, reminiscent of the dilated endothelial sacs observed in the lesions of patients with CCM1. Using in situ hybridization Whitehead et al.56 demonstrated that the vascular defects in CCM1 mice are associated with the loss of arterial endothelial markers and subsequent failure of smooth muscle recruitment to the developing arteries. They found that the expression of the arterial-specific mammalian NOTCH genes (DLL4 and NOTCH4) was significantly downregulated in CCM1-/- embryos before the gross appearance of the mutant phenotype. Given the observed similarities between the enlarged vessels in CCM1–/– embryos and human CCMs, the authors speculated that a similar disruption of arterial identity might underlie the pathology of cavernous malformations in patients with CCM1. Using antibodies against human NOTCH4, they examined previously excised cavernous malformations from three patients with familial CCM1. They were unable to detect NOTCH4 expression in the cavernous malformations. NOTCH4 expression was also reduced markedly in the arteries of the brain tissue adjacent to the vascular malformation. Control preparations from unaffected individuals showed prominent expression of NOTCH4 in arterial endothelium and smooth muscle cells. Because CCM1 is an autosomal dominant disease in humans, it was expected that heterozygous knockout mice would be an appropriate model for the disease. However, careful screening over multiple generations revealed no vascular abnormalities in the brains of CCM1+/- animals. As mentioned, complete loss of CCM1 (CCM1–/–) from a defect in arterial morphogenesis is lethal in midgestation. These findings have led to speculation that the formation of cavernous malformations requires a somatic loss of the wild-type allele. In sporadic cases of CCM, two independent somatic mutations would be required in the same cell, whereas in the familial form only one somatic mutation would be required. This two-hit model would explain the focal, randomly scattered nature of the lesions in patients with the familial form of the disease. In support of this hypothesis, Plummer et al.57 have recently reported that loss of the tumor suppressor gene p53 sensitized mice with the CCM1 mutation to form cerebral vascular malformations. The authors crossed mice that were heterozygous for CCM1 and homozygous for loss of the tumor suppressor gene p53, which has been shown to increase the rate of somatic mutations. They found cerebral vascular malformations that were double mutants (CCM1+/- p53–/–) in 55% of animals, but not in other genotypes. Pathologically, the vascular lesions had many of the characteristics of cavernous malformations. Importantly, loss of p53 alone (CCM1+/+ p53-/-) was not associated with development of vascular malformations. In nature, a single phenotype may arise from mutations of multiple genes that converge on a common cellular pathway. Hereditary hemorrhagic telangiectasia (HHT) is a well-documented example of a vascular disease in which mutations involving three separate genes produce a common phenotype by three coded proteins that form a heteromeric complex in the same signal transduction pathway.58 Likewise, multiple genetic loci have been identified in families with CCMs and are suspected to converge on a common signaling pathway. In 2003, Liquori et al.59 identified the CCM2 gene at 7p13 as MGC4607, a known gene of unknown function, and named it malcavernin. Eight mutations were identified in nine families with one shared mutation. The mutations, which map throughout the MGC4607 gene, include five frameshift, one nonsense, and two splicing mutations, each of which would result in a truncated protein. MGC4607 was selected as a potential candidate for mutation sequencing because its translated protein product encoded a phosphotyrosine-binding (PTB) domain. This same domain is found in ICAP-1α, a binding partner of the CCM1 gene product KRIT1. Subsequent work by Denier et al.60 confirmed identification of MGC4607 as the CCM2 gene in an additional 10 families. In this second study, mutations in the MGC4607 gene included two large deletions and eight additional point mutations. The MGC4607 gene has been reported in several genome databases, but little is known about its function. It extends over 76 kb and includes 10 coding exons. The coding portion of the cDNA is 1323 bp long and encodes for a 444-amino-acid predicted protein. MGC4607 is highly expressed in skeletal muscle, heart, liver, and brain. The presence of a PTB domain in MGC4607 predicts a possible interaction with the CCM1 protein, KRIT1, or with β1-integrin. MGC4607 may complete with ICAP-1a to regulate β1-integrin signaling. Bergametti et al.61 recently identified the CCM3 gene at 3q26.1 as PDCD10 (programmed cell death 10). Seven distinct deleterious mutations were identified in seven families. There were three nonsense mutations, three splicing mutations, and one large deletion. PDCD10, also known as TRAF15, was initially identified by screening for genes differentially expressed during the induction of apoptosis in the TF-1 premyeloid cell line. This gene is upregulated in a fibroblast cell line exposed to specific apoptosis inducers.62 Its cDNA extends over 50 kb and includes seven coding exons and three 5′ noncoding exons. Three alternative transcripts encoding the same protein, differing only in their 5′ untranslated regions (UTRs), have been identified for this gene. The coding portion of the cDNA is 636 bp long and encodes a 212-amino-acid predicted protein. Searches of the protein databases with the coding sequence of Homo sapiens PDCD10 failed to reveal any known transmembrane or functional domains. Northern blot analysis of human tissues using the entire cDNA as a probe revealed ubiquitous expression in all tissues except colon.61 These preliminary data suggest that this gene, which is highly conserved from invertebrates to humans, plays a role in apoptotic pathways. The role of this protein in angiogenesis and its link to KRIT1 and MGC4607 remain to be investigated. Initial estimates suggested that 40% of individuals with familial CCM might be linked to the CCM3 locus,23,63 but recent data indicate that this number is too high. In a report by Bergametti et al.61 identifying the CCM3 gene, mutations in the PDCD10 gene were identified in only 8 to 20 families with the CCM phenotype. Because the 20 families in this study were included on the basis of a negative screening for CCM1 and CCM2 mutations, a higher proportion of PDCD10 mutations would have been expected. This observation is supported by additional research from Doug Marchuk’s group at Duke (D. Marchuk, personal communication, 2005) who found a CCM3 mutation in only 10% of families without a CCM1 and CCM2 gene mutation. Although several hypotheses can be raised to explain this discrepancy, the authors concluded that the possibility of a fourth CCM gene cannot be excluded. Familial cavernous malformations result from mutations affecting at least three distinct loci. Based on available data, at least two of these loci (CCM1 and CCM2) appear to be involved in the regulation of β1 integrin signal transduction, which plays a crucial role in the regulation of cell adhesion and migration during angiogenesis. The third locus (CCM3) involves an apoptotic pathway. The role of the latter in angiogenesis and its link to KRIT1 and MGC4607 in the formation of cavernous malformations remains to be investigated. 15. Gunel M, Awad IA, Anson J, Lifton RP. Mapping a gene causing cerebral cavernous malformation to 7q11.2-q21. Proc Natl Acad Sci U S A 1995;92(14):6620–6624 49. Rupp PA, Little CD. Integrins in vascular development. Circ Res 2001;89(7):566–572
Genetics of Cerebral Cavernous Malformations
CCM1
CCM2
CCM3
CCM4
Conclusion
References
< div class='tao-gold-member'>
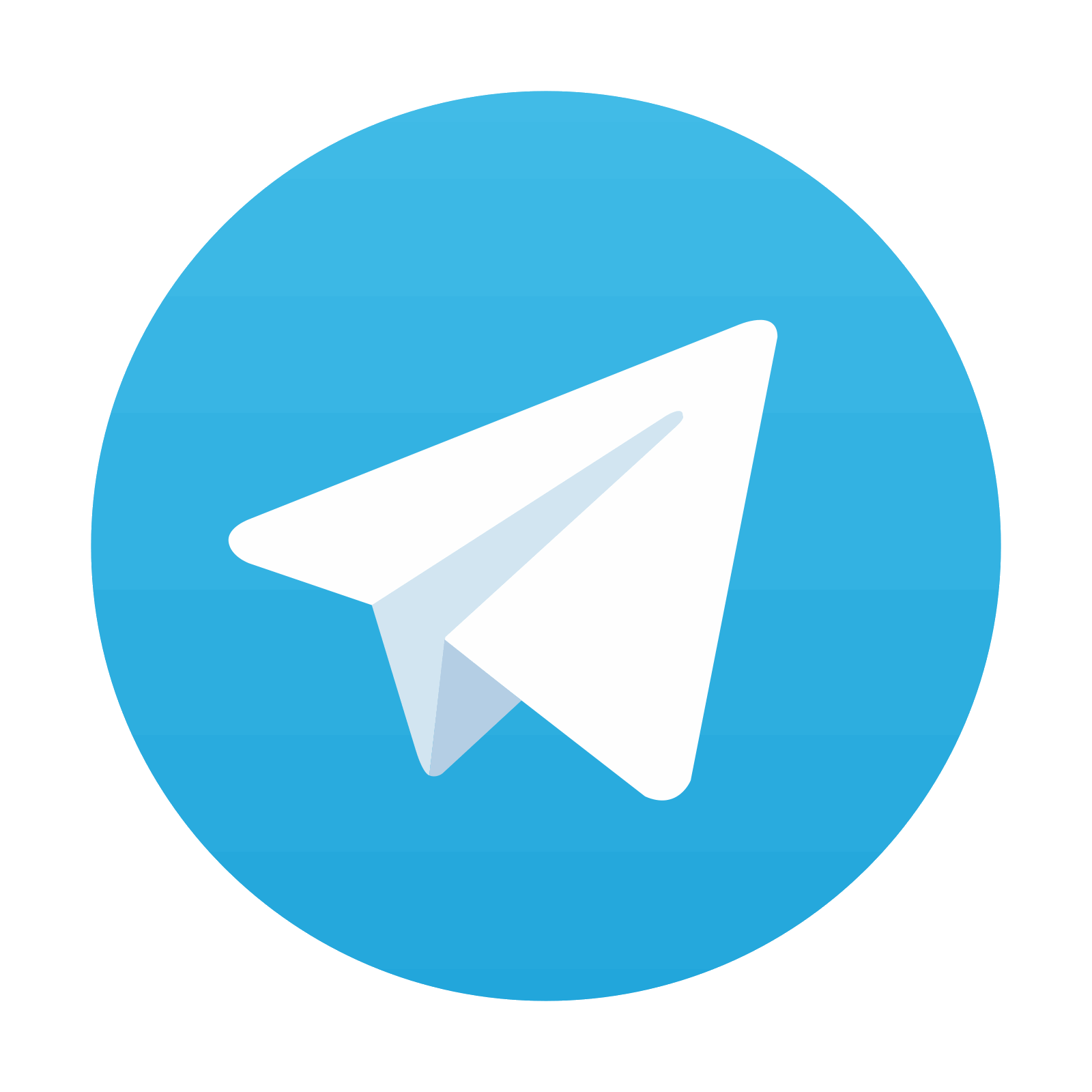