Clinical characteristics associated with the major FTLD genes GRN, C9orf72, and MAPT. Shaded areas represent interquartile distribution of onset age (y-axis) and FTLD subtype (x-axis). Vertical lines represent standard deviations of onset ages. Based on AD & FTLD Mutation Database [7].
GRN
Despite extensive mutation analyses, a MAPT mutation could not be identified in all FTLD families linked to chromosome 17q21. When it became apparent that in these FTLD families the ubiquitin-positive inclusions were tau-negative, a different genetic cause of disease was anticipated. Mutation analyses of other genes in the chromosome 17q21 region identified mutations in GRN, located 1.5 Mb centromeric of MAPT [16, 17]. To date, 69 different GRN mutations have been reported in 264 families (Table 14.1) [7]. GRN encodes progranulin, a ubiquitously expressed growth factor precursor which is processed into 7.5 granulin peptides [14]. Both full-length progranulin and the granulin peptides are implicated in a wide range of biologic processes such as inflammation and wound repair, as well as in pathologic conditions including tumorigenesis. Although the roles of progranulin and the processed granulin peptides in the central nervous system are not well established, in vitro and in vivo studies suggest a neurotrophic function involved in neuronal survival and neurite outgrowth [18]. GRN mutations linked with FTLD lead to the loss of one functional allele. Most mutations produce null alleles as a result of mRNA decay mediated by a nonsense mutation, or frameshift mutation introducing a premature termination codon. Other loss-of-function mechanisms including gene deletion, mutations interfering with protein sorting, and mutations disrupting protein structure were reported. As a result, these mutations cause FTLD through a haploinsufficiency mechanism [14].
The functional impact of GRN missense mutations and their association with disease is less straightforward: some compromise protein stability or cellular sorting and may result in loss of function. Examples of such loss-of-function missense mutations are those disrupting the signal peptide or the cysteine disulfide bonds controlling the characteristic granulin peptide fold. Other missense mutations are more likely to partially compromise granulin function and behave as risk alleles [14]. GRN missense mutations have been suggested to be rare risk variations in Alzheimer’s disease (AD) [19, 20], and ALS [21]. Using circulating granulin levels in cerebrospinal fluid, serum, or plasma as a protein biomarker may be an informative discriminator between benign and risk variations.
All GRN-associated FTLD patients develop a FTLD-TDP proteinopathy type A, characterized by numerous short dystrophic neurites (DN) and neuronal cytoplasmic TDP-43 inclusions (NCI) in the superficial cortical layers of the brain (see Chapter 13). Of the FTLD-TDP type A patients, GRN mutations explain about 40% [22]. Despite the fact that haploinsufficiency is the common disease mechanism in all patients carrying a GRN mutation, the associated clinical phenotype is variable, including bvFTD and PNFA [23]. Clinical diagnoses of related neurodegenerative diseases, including AD and parkinsonian disorders, have been associated with GRN mutations [23, 24]. A clinical review of 97 GRN mutation carriers noted a diagnosis of AD in 11% and parkinsonian symptoms and motor neuron symptoms were each observed in 5% of GRN mutation carriers [25]. A literature survey of 183 patients with GRN-associated FTLD demonstrated that the mean onset age was 61 ± 9 years, i.e., 13 years later than in MAPT mutation carriers (Figure 14.1) although, as in FTLD-tau, there is a wide distribution of onset ages, ranging from 35 to 87 years [7, 14]. Age-dependent penetrance was observed, with 50–60% of mutation carriers being affected by 60 years and 90–95% by age 70 years [26], resulting in an often unrecognized family history. Consequently up to 25% of patients with a GRN mutation are diagnosed with sporadic FTLD [25]. In this group of patients, GRN mutations explain 3%.
C9orf72
Although signs of ALS occur in as much as 15% of FTLD patients, neither MAPT nor GRN mutations were associated with FTLD-ALS. In such families segregating FTLD and ALS, genome-wide linkage studies identified a disease locus at chromosome 9p13–p21 [27, 28]. Since the first identification of this locus, more than 15 autosomal dominant families with ALS and FTLD worldwide were genetically linked to the same chromosomal region, suggesting a major disease locus for FTLD-ALS. Meanwhile, genome-wide association studies in ALS patients of European origins demonstrated that the same chromosomal region harbored a genetic risk factor for ALS, FTLD, and ALS-FTLD. In a Finnish ALS study the susceptibility region was narrowed to a 232 kb genomic segment containing three protein-coding genes [29]. The genomic region shared between the FTLD linkage and ALS association studies and the extensive mutation analyses of all genes in that region resulted in the identification of a pathologically expanded GGGGCC hexanucleotide repeat in the regulatory region of C9orf72 associated with FTLD and ALS [30–32]. In Western Europe and North America, the normal GGGGCC repeat size is smaller than 25 units, while in Asian populations the normal repeat size range does not exceed 15 units [33]. In contrast, the size of pathologically expanded repeat alleles is generally larger than 60 repeat units [33] and can extend to over 4000 repeat units.
Numerous studies worldwide confirmed that the GGGGCC repeat expansion in C9orf72 is the most common cause of diseases in the FTLD-ALS continuum. Globally, frequencies are the highest in White populations of Europe and North America, ranging from 3.5% to 18% in FTLD (Table 14.1), 7% to 28% in ALS, and 15% to more than 50% in FTLD-ALS [33]. In European populations, frequencies were markedly elevated in ALS patients of Finland, Sweden, and Denmark. In strong contrast, pathologic repeat expansions were rarely observed in Asian populations, with frequencies of 0.4–4.8% in ALS [34]. Based on these observations and the fact that the repeat expansions occur on the same haplotype, some studies suggested a common northern European founder [35]. In contrast, a detailed study rather supported multiple independent expansion events on the same haplotype, possibly harboring repeat-destabilizing elements [36]. Patients with a family history of disease showed 3 to 12 times higher frequencies compared with non-familial patients. Nevertheless, especially in ALS, where family history is observed in only 10% of patients, sporadic ALS patients carrying an expanded repeat outnumber familial ALS patients. In both, FTLD (AD & FTLD Mutation Database [7]) and ALS (ALS Online Genetics Database [37]), the frequency of C9orf72 repeat expansions significantly exceeded that of any other individual mutation causing disease. The highest mutation frequencies were recorded in familial patients with combined FTLD and ALS symptoms.
C9orf72 encodes a ubiquitously expressed protein of unknown function. It is expressed as three major transcripts which are translated in two different protein isoforms. The expanded GGGGCC repeat is located in the non-coding, proximal regulatory region of C9orf72 [33]. Pathologic repeat expansions result in near-complete loss of expression of the three major gene transcripts [30–32], suggesting that haploinsufficiency contributes to the disease mechanism. In addition, accumulation of transcripts harboring the expanded GGGGCC repeat in nuclear RNA foci was described [30, 38]. Detailed studies of such RNA foci in other repeat expansion diseases such as Huntington’s disease and spinocerebellar ataxias have demonstrated that they mediate a toxic gain of RNA function. In this mechanism, RNA species containing expanded repeats bind select RNA-binding proteins (RBPs), thereby disrupting their normal function. Interestingly, GGGGCC repeats were shown to bind heteronuclear RNA-binding protein A3 (hnRNPA3), which accumulates in neuronal cytoplasmic and intranuclear inclusions in the hippocampus [39]. Recently, the expanded repeat sequences were also demonstrated to be transcribed into abnormal sense and antisense RNA species. In addition to potentially contributing to the formation of RNA foci, they were actively translated into five different dipeptide repeats (DPRs), depending on the reading frame [40]. These DPRs were shown to be a component of the star-shaped intraneuronal aggregates/inclusions in the hippocampus, cerebellum, and neocortex, specific to C9orf72 expansion carriers.
Neuropathologically, FTLD-C9orf72 is generally associated with TDP-43 proteinopathy type B, characterized by NCI throughout the cortex, hippocampus, and in some patients also in motor neurons and spinal cord (see Chapter 13). Motor neurons and spinal cord involvement were associated with clinical signs of MND [38]. However, the number and distribution of TDP-43 inclusion pathology was variable and in some patients more consistent with a TDP-43 type A [41] or FTLD-ubiquitin proteasome system (UPS), lacking detectable TDP-43 neuropathology [32, 42]. Abundant star-shaped, p62-positive and TDP-43-negative NCI, and rare neuronal intranuclear inclusions (NII) were seen in the pyramidal cell layer of the hippocampus and cerebellar granular layer of most patients. These inclusions were shown to be highly specific for the C9orf72 mutation carriers and contain DPRs translated from the expanded hexanucleotide repeats as well as RBPs, including hnRNAP3 [39, 40].
Patients with a C9orf72 repeat expansion clinically present with widely variable symptoms of the FTLD-ALS spectrum including FTLD, ALS, and FTLD-ALS [33]. Independent of concomitant ALS, FTLD is mostly of the behavioral type but patients with PNFA have been described. The mean onset age is 57 years [34, 43], which is intermediate to the mean onset ages in FTLD associated with MAPT and GRN (Figure 14.1). Also here, a high variability in onset age was noted, ranging between 30 and 76 years [34]. Typical of repeat expansion diseases, genetic anticipation has been suggested with a 7-year decreased onset age in the two succeeding generations [44]. However, inverse correlation of repeat expansion size and onset age needs to be demonstrated.
VCP
Linkage analysis and subsequent mutation analyses in autosomal dominant families with a multisystem proteinopathy characterized by inclusion body myopathy (IBM), Paget’s disease of the bone (PDB), and FTLD (IBMPFD) identified mutations in VCP at chromosome 9p13 [45]. Today, 19 different mutations have been identified in 49 independent families (AD & FTLD Mutation Database [7]). VCP encodes a ubiquitously expressed member of a family of AAA-ATPases associated with many cellular functions through interactions with different adaptor proteins. All IBMPFD mutations affect amino acid residues located at the interface between the D1 ATPase and the CDC48-like N domain of the protein [46]. The best supported hypotheses of the disease mechanism of VCP mutations are disturbed ubiquitin-mediated proteasomal protein degradation [47] and autophagy, possibly acting in a concerted fashion [48].
FTLD patients with a VCP mutation are associated with TDP-43 proteinopathy type D, characterized by large numbers of NCI, NII, and DN in affected neocortical regions [5]. Some inclusions also stain for VCP protein p97 [49]. Symptoms of FTLD due to a VCP mutation usually present in the mid-50s in 25–30% of IBMPFD patients [45]. Because the symptoms of the IBMPFD syndrome display incomplete penetrance, patients may present with classical FTLD. Nevertheless, in classical FTLD, VCP mutations are rare and represent less than 1% of familial FTLD (Table 14.1). The most frequently reported FTLD subtypes associated with a VCP mutation are bvFTD and SD [50]. Interestingly, motor neuron involvement was reported in IBMPFD and VCP mutations were reported in 1–2% of ALS patients [51].
CHMP2B
In a Danish FTLD family with particular neuropathologic features characterized by vacuolar abnormalities in cortical neurons and negative for tau, TDP-43, and FUS antibodies, linkage analyses identified a mutation in CHMP2B at chromosome 3p11.2 [52]. Subsequent mutation analyses identified four mutations in five families (Table 14.1) (AD & FTLD Mutation Database [7]). The mutation of the Danish family and a more recently identified nonsense mutation in exon 5 of 6 were both shown to result in C-terminal protein truncation, by aberrant exon splicing [52] and introducing a premature termination codon [53], respectively. Nevertheless, missense mutations were also reported. CHMP2B encodes a component of the heteromeric ESCRT-III (endosomal sorting complex required for transport III) complex with functions in the endosomal–lysosomal and the autophagic protein degradation pathway. The gene is expressed in neurons of all major brain regions. CHMP2B mutations were associated with enlarged vacuoles in neurons in the frontal, temporal, parietal, and occipital cortices, due to impaired endosome–lysosome fusion [54], and autophagy [55]. Ubiquitin-immunoreactive NCI do not stain for tau or TDP-43 antibodies, consistent with a pathologic classification of FTLD-UPS [2].
The clinical diagnosis of most patients in the Danish CHMP2B family was bvFTD, with early personality changes being the most prominent feature. In other patients, progressive aphasia involvement was described, although diagnostic criteria for PNFA, SD, or logopenic aphasia were not met. The aphasia is characterized by a reduction in spontaneous speech or mutism, while reading and repetition was conserved. The average onset age is 58 years, ranging between 46 and 65 years [53, 56]. Interestingly, rare CHMP2B mutations were also reported in ALS patients [55].
Mendelian ALS genes TARDBP and FUS
Mutations in TARDBP and FUS are associated with ALS. TARDBP mutations were initially identified as a direct consequence of the occurrence of TDP-43-derived protein species as the major constituent of the aggregates in upper and lower motor neurons of superoxide dismutase 1 (SOD1)-negative ALS patients and in FTLD-U patients [3, 4]. Subsequently, TARDBP mutations were identified in up to 5% of familial ALS patients. Also, in FTD-ALS and in FTLD without signs of motor neuron involvement, mutations were found [57], although here the mutation frequency is below 1% (Table 14.1).
TDP-43 is an RNA-binding protein that forms heterogeneous nuclear ribonucleoprotein complexes (hnRNP) which function in RNA processing activities, including transcription, RNA splicing, and microRNA processing. Missense mutations were found in the C-terminal glycine-rich region involved in protein–protein interactions.
Similar to TARDBP, FUS is a member of the family of hnRNP proteins. Its location in the chromosome 16p11.2 region, previously linked to ALS, made it an excellent candidate gene. Mutation analyses of FUS in patients of 16p11.2-linked families and other ALS families identified FUS mutations [58]. Subsequently, FUS mutations were also detected in FTLD, although they are rare and their role in FTLD genetics remains to be established [59].
Interestingly, an accumulation of FUS protein in inclusion bodies in neuronal cytoplasm and nucleus was associated with three clinicopathologic subtypes of tau-negative, TDP-43-negative FTLD, defined by distinct characteristics and location of NCI and NII (FTLD-FUS) [60]. FUS pathologies are often associated with particular, rapidly progressing FTLD with a strong behavioral component. Atypical FTLD-U (aFTLD-U) usually has a very early-onset age in 30s or 40s and a disease duration of approximately 7 years [61]. Patients with neuronal intermediate filament inclusion disease (NIFID) usually develop the disease between 40 and 60 years of age and disease duration is on average approximately 3 years [61]. Finally, also patients with basophilic inclusion body disease (BIBD) show FUS immunoreactivity in frontal cortex, basal ganglia, and brainstem [60], and present with a clinical syndrome of early-onset ALS, occasionally accompanied with bvFTD [60].
Susceptibility genes and risk loci in FTLD
TMEM106B
A GWAS in 515 FTLD-TDP patients resulted in the identification of multiple single nucleotide variations (SNPs) located in a region at chromosome 7p21 containing the gene TMEM106B [9]. The finding was replicated in subsequent association studies [62]. The 7p21 risk haplotype was suggested to lead to increased expression of TMEM106B in the brain [9], although this was not replicated in another study [63]. It was suggested that TMEM106B may affect onset age in FTLD associated with a GRN mutation by increasing granulin levels [64], possibly by modulating brain connectivity as demonstrated in asymptomatic GRN mutation carriers [65]. TMEM106B was also associated with cognitive impairment in ALS [66]. Interestingly, TMEM106B was also reported to be a genetic modifier in C9orf72 repeat expansion carriers [67] by alleviating the severity of FTLD manifestation, but not of motor neuron deficits [68].
TMEM106B is a type 2 integral membrane protein localizing to late endosomes and lysosomes [69]. It may induce morphologic changes of lysosome compartments and delay the degradation of endocytic cargoes by the endolysosomal pathway [70]. A recent study demonstrated that it interacts with microtubule-associated protein 6 (MAP6) to control dendritic trafficking of lysosomes [71]. Interestingly, it was shown that inhibition of vacuolar H+-ATPases results in increased levels of both TMEM106B and granulin, providing a potential biochemical link [69].
Risk variations in Mendelian FTLD genes
Besides harboring Mendelian FTLD mutations, MAPT was associated with risk of PSP, CBS, and PD, but inconsistent results were found in FTLD [13]. MAPT is represented in the human population as two genetically distinct haplotypes H1 and H2 owing to its location inside a commonly inverted genomic region [72]. The H1 haplotype is overrepresented in the 4R tau disorders PSP and CBD. In young PSP patients, the risk was in part attributed to a SNP located in the large first intron of MAPT, which potentially modulates tau expression by modifying a LBP-1c/LSF/CP2-binding site [73]. A two-stage GWAS in 2165 PSP patients confirmed and extended these findings, and further implicated STX6, EIF2AK3, and MOBP in PSP [74]. Together these genes suggested roles of vesicle–membrane fusion at the Golgi–endosomal interface, the endoplasmic reticulum unfolded protein response in PSP.
In a series of pathologically confirmed FTLD-U patients without GRN mutations, a common genetic variant rs5848 located in a microRNA-binding site in the 3′-untranslated region (UTR) of GRN was identified as risk factor for FTLD-U [75]. A significant reduction of granulin protein was observed in homozygous T-allele carriers in vivo, suggesting a similar mode of action to heterozygous loss-of-function mutations in GRN. Another variant in the first intron of GRN, potentially affecting its expression, was also reported to be associated with FTLD in another patient series [76]. Nevertheless, subsequent studies could not confirm genetic risk for FTLD associated with GRN variants. Further, promoter methylation plays a role in GRN expression and is altered in FTLD brain [77].
Pathologically expanded C9orf72 repeat sizes usually exceed 60 units. However, the normal repeats less than 25 units were shown to have a size-dependent negative effect on gene expression [78]. Although the impact of C9orf72 expression on development of disease remains unclear, it is of interest to note that genetic association was reported between the longer repeats within the normal size range and FTLD [78].
Concluding remarks
The clinical, pathologic, and genetic heterogeneity of FTLD has long impeded research aiming to understand underlying disease mechanisms. The strong genetic component of FTLD has significantly contributed to elucidate different aspects of this condition. We now have knowledge of the most frequently mutated genes involved in the pathogenesis of FTLD, i.e., GRN, C9orf72, and MAPT. These are associated with distinct neuropathologic hallmarks, i.e., MAPT leads to FTLD-tau, and GRN and C9orf72 are both associated with a FTLD-TDP proteinopathy, although they are each associated with distinct neuropathologic characteristics. Also, the identification of C9orf72 has exposed novel types of inclusions resulting from expanded GGGGCC repeats. The currently known FTLD genes explain together up to 65% of familial FTLD (Table 14.1), leaving at least 35% of familial FTLD genetically unexplained. While large autosomal dominant FTLD families are available to discover additional FTLD genes, it is unlikely that other high-frequency genes will be identified. Current sequencing technologies like whole-exome and -genome sequencing will most likely identify private genes and mutations in one or a few families. Nevertheless, these rare genes can provide further insight into the pathobiology of FTLD by identifying new molecular pathways or marking key proteins in known biologic pathways.
The search for risk and protective genes for FTLD has also been greatly impeded by the clinicopathologic and underlying genetic heterogeneity. Especially, the relatively weak correlation between clinical diagnosis and underlying genetic etiologies is complicating strategies to define inclusion criteria to maximize the power of GWASs by enriching for a limited number of susceptibility genes. One GWAS used TDP-43 proteinopathy to select a more homogeneous study cohort [9], a strategy which resulted in the identification of TMEM106B, the first common risk gene for FTLD. Other susceptibility genes can be expected especially in SD, where family history is much less prominent than in bvFTD and PNFA. Consistent with this observation, mutations in the known FTLD genes are extremely rare in SD. Interestingly, SD is associated with a distinct type C of TDP-43 proteinopathy (see Chapter 13). FTLD-TDP type C is characterized by the presence of long DN localized in the superficial cortical layers, while NCI and NII are rare or absent compared with the other subtypes. Together, these observations might suggest that SD is clinically, pathologically, and genetically distinct from other types of FTLD and might predominantly be caused by the interaction of multiple yet unknown risk and protective genes.
The wide range of clinical diversity associated with the Mendelian FTLD genes, i.e., onset age, FTLD clinical subtype, and motor neuron involvement, is not understood. Genetic as well as environmental modulating factors are likely to influence onset and pres-entation of the disease. TMEM106B was suggested to increase granulin protein expression and to delay disease onset. It may also modulate cognitive involvement in C9orf72 repeat expansion carriers in the FTLD-ALS diseases. However, TMEM106B cannot explain the complete variance, and other modifier genes must exist. Linkage-based modifier screens in extended families and association-based studies in cohorts of patients with extreme clinical phenotypes due to the same genetic type of disease may reveal such additional modifier genes. Onset age-modifying genes might be interesting therapeutic targets, aiming at delaying onset of clinical symptoms.
With the identification of an increasing number of disease-associated genes, common disease pathways may emerge. Again the heterogeneity of FTLD suggests that a number of distinct pathways act in concert to initiate processes leading to different proteinopathies. Whether the distinct classes of FTLD-TDP proteinopathies share common downstream disease mechanisms is questionable. The fact that C9orf72 repeat expansions are associated with both FTLD-TDP type B and type A, and that TMEM106B may play a role in both pathologic subtypes, suggests that the disease mechanisms of at least these FTLD-TDP subtypes converge in common pathways. Disrupted RNA and protein homeostasis and autophagy processes may represent such pathways [79]. The identification of additional rare Mendelian FTLD genes and common susceptibility genes will be of great use to further define this and other pathways.
In conclusion, recent molecular genetics’ breakthroughs have been at the basis of our growing understanding of the pathobiology of FTLD and related disorders. These emerging disease mechanisms will facilitate progress in the development of therapeutic strategies. Continued efforts to identify additional Mendelian, risk, and modifying genes will further accelerate the progress into downstream translational research. In this respect, the increasing impact of novel high-throughput molecular genetic technologies promises exciting times to come in the research of these neurodegenerative diseases.
Note
While production of this chapter was in progress, a novel Mendelian FTD gene, i.e. TANK-binding kinase 1 (TBK1) was discovered. TBK1 was first identified as a novel risk gene for ALS, in which predicted damaging non-synonymous variants were significantly enriched in the exomes of > 2800 ALS patients [81]. In another exome sequencing study, TBK1 loss-of-function mutations were identified in 9 of 252 genetically unexplained familial ALS patients, but not in > 800 control exomes [82]. Clinical evaluation of the families of the latter study indicated cognitive impairment, often progressing to FTD, in 50% of patients. A subsequent genome sequencing study of 104 FTLD-TDP patients negative for C9orf72 and GRN mutations revealed four TBK1 variations including one nonsense and three missense mutations, all with predicted damaging effect on TBK1 function [83]. Among the mutation carriers were two patients with FTLD in the absence of ALS symptoms. A targeted mutation analysis of TBK1 in a hospital-based cohort of 482 unrelated FTD and FTD-ALS patients and 147 ALS patients in Belgium identified 11 loss-of-function (LOF) mutation carriers, resulting in a mutation frequency 1.1% in FTD (5∕460), 4.5% in FTD-ALS (1∕22) and 3.4% in ALS (5∕147) [84]. For several mutations, including mutations introducing a premature termination codon, in-frame deletions, and missense mutations, loss of transcript and protein was demonstrated, suggesting that the complete mutation spectrum can result in loss of TBK1 function [83, 84]. Although large genetic epidemiologic studies have not been reported yet, these early findings suggests that TBK1 is the third most prevalent FTD gene after C9orf72 and GRN, and the second most common FTD-ALS gene after C9orf72. Reported onset ages are between 61 and 86 years with an average of 70 years [83, 84], which is the latest of all genetic subtypes of FTD and FTD-ALS today. Pathological examinations in seven families demonstrated FTLD-TDP type B in four and type A in three families [82–84]. Given the limited information available today, it is unclear whether TBK1 mutations can result in different pathologic FTLD-TDP subtypes, potentially modulated by other genetic factors. Alternatively, pathology data may be contaminated with observations derived from carriers of neutral TBK1 variants with unproven loss-of-function effect.
TBK1 is an IKK-related serine/threonine protein kinase with multiple targets. It has been implemented in many biological functions including innate immune response/inflammation, autophagy, and cell proliferation. Among its targets are optineurin (OPTN) and p62, two other genes that play a role in autophagy and inflammation that have been implicated in the genetics and pathobiology of the ALS-FTD diseases [81]. Together, these findings further corroborate the central roles of these processes in FTD and ALS.
Acknowledgments
The research performed by the authors was in part funded by the Belgian Science Policy Office Interuniversity Attraction Poles Programme; the Flemish government-initiated Methusalem Excellence Program; the Alzheimer Research Foundation; the Medical Foundation Queen Elisabeth; the Research Foundation Flanders; the Agency for Innovation by Science and Technology Flanders; the University of Antwerp Research Fund, Belgium; and the MetLife Foundation (USA) Award for Medical Research to C.V.B.
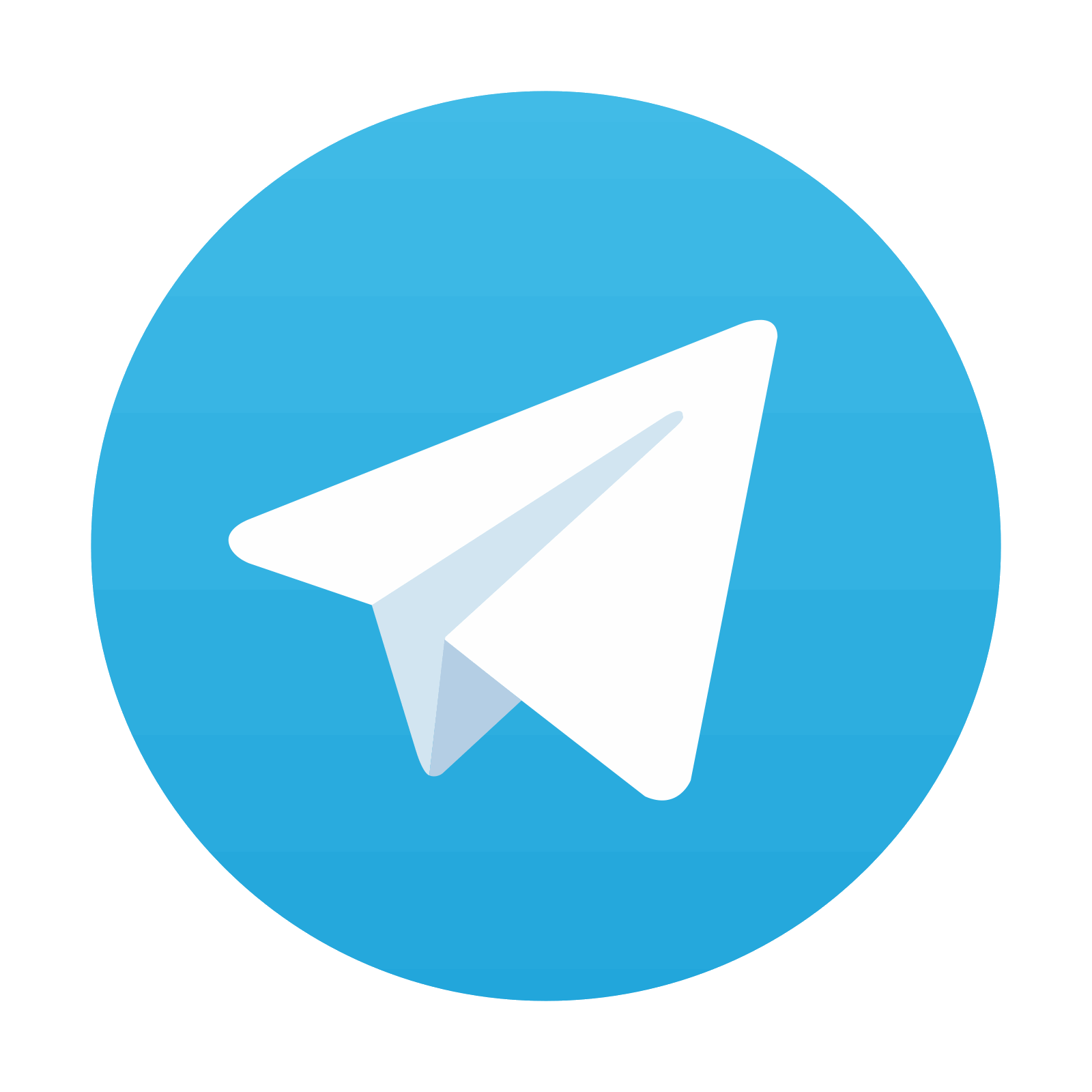
Stay updated, free articles. Join our Telegram channel
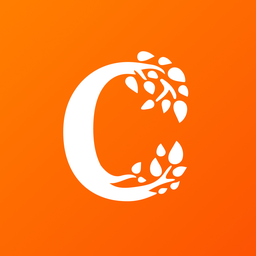
Full access? Get Clinical Tree
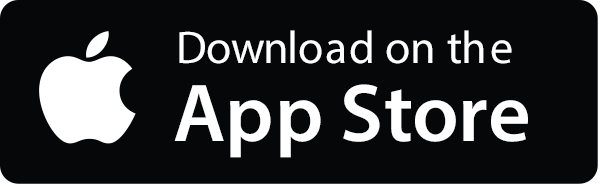
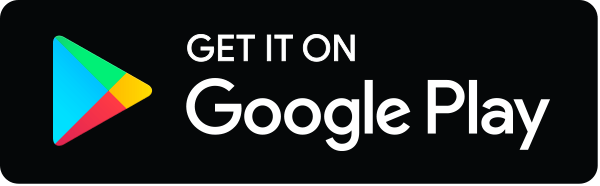