Fig. 4.1
Schematic depiction of glial glutamate and lactate transporter functions. a (1) Presynaptic neuronal impulses induce glutamate (Glu) release into the synaptic cleft. Glutamate binds and opens postsynaptic AMPA and NMDA receptors leading to enhanced Ca2+ influx and propagation of the presynaptic stimulus to the postsynaptic neuron. (2) To limit excessive postsynaptic stimulation, glutamate is taken up by astrocytes through GLT1 transporters. This process is coupled to Na+ uptake and increased Na+/K+ pump activity leading to an increased metabolic demand, i.e. the production of ATP. (3) To enhance ATP generation, glucose is taken up from blood vessels into the astrocytes through glucose transporters (Glut1). The astrocytes convert glucose to pyruvate and lactate, generating ATP through glycolysis. (4) Lactate, the end product of glycolysis could diffuse through connexins (Cx) to oligodendrocytes. Alternatively oligodendrocytes might generate lactate through glycolysis after glucose uptake from the blood stream. b (5) Oligodendrocytes export lactate through MCT1 transporters upon which neurons can import lactate through MCT2 transporters and use as a metabolic substrate
Here, we briefly outline functions of these glial transporters (Fig. 4.1). As action potentials invade presynaptic neuronal terminals, glutamate gets released into the synaptic cleft, where it binds and opens postsynaptic α-amino-3-hydroxy-5-methyl-4-isoxazolepropionic acid (AMPA) and N-methyl D-asparate (NMDA) ionotropic glutamate. To limit excessive postsynaptic stimulation, glutamate is taken up by astrocytes through plasma membrane glutamate transporters, a process coupled to Na+ uptake and increased Na+/K+ pump activity leading to an increased metabolic demand, i.e. the production of ATP. To enhance ATP generation, glucose is taken up from blood vessels into the astrocytes through glucose transporters. The astrocytes convert glucose to pyruvate and lactate , generating ATP through glycolysis. Lactate, the end product of glycolysis could diffuse through gap junctions to oligodendrocytes . Alternatively, oligodendrocytes might generate lactate through glycolysis after glucose uptake from the blood stream. Oligodendrocytes export lactate through MCT1 transporters upon which neurons can import lactate through lactate transporters and use as a metabolic substrate.
4.2 Glutamate Transporters
The maintenance of low extracellular levels of the primary excitatory neurotransmitter glutamate at the neuronal synapse is crucial to ensure fast and efficient excitatory synaptic transmission in the CNS, particularly because there is no evidence for extracellular metabolism of glutamate . To be able to rapidly return to these homeostatic levels of glutamate after synaptic firing, plasma membrane spanning glial and neuronal high affinity glutamate plasma membrane transporters remove any excess glutamate via a sodium-potassium coupled uptake mechanism (Fig. 4.1a). Any unwanted rise in extracellular glutamate above normal leads to pathological activation of neuronal glutamate receptors, which in turn triggers intracellular events in the postsynaptic neuron leading to neuronal cell death. This phenomenon is known as glutamate excitotoxicity and is the underlying mechanisms of a number of neurodegenerative as well as neuropsychiatric diseases. A loss of functional plasma membrane glutamate transport has been suggested to contribute significantly to excitotoxic neuronal cell death and regulation of glutamate transporters, and consequently the synaptic glutamate homeostasis, has therefore been an increasing interest in therapeutic development for neurological disorders .
4.2.1 Structure and Expression
Initial structure and function studies on EAATs have been based on molecular pharmacology approaches (Danbolt 2001). The recent crystallization of a prokaryotic orthologue GltPh(Yernool et al. 2004) however provided an enhanced understanding into the structural details of EAATs as well as transport mechanisms (Boudker et al. 2007). The novel crystal structure predicted the presence of eight transmembrane domains and two re-entrant hairpin loops, with both, N- and C-terminal intracellular domains. While there is general agreement that the information obtained from the GltPh will be informative for the structure and function of mammalian EAATs, future studies are needed to confirm commonality between these two transporter orthologues in regards to membrane topology, substrate preference, etc. (for a detailed review on glutamate transporter structure and function see (Jiang and Amara 2011)). Clearly, understanding the detailed mechanisms of neurotransmitter transport and potential conformational changes during this process will be important for the ongoing development of therapeutic compounds targeting these transporters.
Five plasma membrane glutamate transporter subtypes have been cloned and characterized thus far: EAAT1-EAAT5 (human nomenclature) or GLAST/GLT-1/EAAC1/EAAT4/EAAT5 (rodent nomenclature, respectively) (Danbolt 2001). The subtypes differ in their expression pattern with regard to cell type and CNS brain region. EAAT2/GLT-1 (Shashidharan et al. 1994) is primarily found on astrocytes and is the major glutamate transporter in the forebrain, estimated to be responsible for over 90 % of functional glutamate transport (Furuta et al. 1997b). EAAT1/GLAST (Shashidharan and Plaitakis 1993) is the major glutamate transporter present on astrocytes in the cerebellum (Furuta et al. 1997b), but also found in the inner ear (Furness and Lehre 1997), the circumventricular organs (Berger and Hediger 2000) and in the retina (Derouiche and Rauen 1995; Rauen 2000). Interestingly, EAAT1/GLAST is the highest expressed glutamate transporter during CNS development, but drops expression levels significantly when adulthood is reached (Furuta et al. 1997a). EAAT3/EAAC1 is a neuronal glutamate transporter with highest concentrations found in the hippocampus, cerebellum and basal ganglia (Furuta et al. 1997a, b). EAAT4 is also a neuronal transporter and for the most part expressed in the Purkinje cells of the cerebellar molecular layer (Barpeled et al. 1997; Furuta et al. 1997a) while neuronal transporter EAAT5 is mainly expressed in rod photoreceptors and bipolar cells of the retina (Arriza et al. 1997).
4.2.2 Physiology
Glutamate transport is facilitated by a co-transport of 2–3 molecules of Na+ and one molecule of H+. The binding of these molecules on the extracellular site of the transporter is thought to induce a conformational change of the protein, which then in turn allows for the counter-transport of one K+, before the transporter resumes its original confirmation (Zerangue and Kavanaugh 1996; Danbolt 2001). This transport cycle allows for the maintenance of a concentration gradient of extracellular versus intracellular glutamate of >10,000 fold. Most importantly, this transport is much slower than rapid excitatory synaptic signaling, allowing for maximum ligand-gated glutamate receptor response at the postsynaptic neuron (Wadiche et al. 1995; Bergles and Jahr 1997) and thereby reinforcing the impact that transporter function has on synaptic activity in the CNS (for reviews see Conti and Weinberg 1999; Huang and Bergles 2004). Experiments using antisense oligonucleotides or targeted gene disruption confirmed the critical role of efficient glutamate transporter activity in the maintenance of a healthy extracellular glutamate homeostasis (Rothstein et al. 1996; Tanaka et al. 1997). Mice lacking the prominent glutamate transporter EAAT2/GLT-1 showed significantly increased extracellular glutamate levels, excitotoxic neurodegeneration and progressive paralysis (Tanaka et al. 1997). While not fatal, knocking down EAAT3/EAAC1 in vivo led to epileptic seizures (Rothstein et al. 1996).
In addition to its role in excitatory neurotransmission, glutamate transport also contributes significantly to the metabolism of glutamate . Glutamate taken up by astrocytes can be recycled via the known glutamate-glutamine cycle: glutamate in astrocytes is converted to glutamine by the enzyme glutamine synthetase . Glutamine is then transported out of the astrocyte and back into neurons by cell type specific glutamine transporters. In neurons, glutamine is converted back to glutamate via glutaminase, where it is then re-packaged into synaptic vesicles and released by exocytosis back into the synaptic cleft (van den Berg and Garfinkel 1971) (for review see Danbolt 2001; Parpura and Verkhratsky 2012). While this metabolic pathway is widely accepted, there is sufficient evidence that glutamate in astrocytes can also be metabolized through different pathways, resulting not only in glutamine, but also lactate , thereby serving as a neuronal energy substrate (see discussion on astrocytic lactate below, also (Danbolt 2001)). In addition, neuronal glutamine is not only converted back to glutamate, but also used for the synthesis of γ-aminobutyric acid (GABA), which makes glutamate ultimately a precursor molecule for the formation of inhibitory GABA-ergic neurons.
4.2.3 Regulation
Given the crucial importance of efficient glutamate uptake activity for the maintenance of the extracellular glutamate homeostasis and prevention of glutamate excitotoxicity, significant efforts have been made to study the regulation of these transporters. Most importantly, any information gained from these studies should facilitate the use of the glutamate transporter system as a pharmaceutical target for the treatment of neurodegenerative diseases that show impaired glutamate uptake activity.
Despite these efforts, the mechanisms of glutamate transporter regulation are still not well defined and only limited factors have been identified that regulate protein expression or transporter activity. The difficulty of identifying specific mechanisms arises from the diversity of glutamate transporter functions, reaching from very fast acute impact on signaling activity at the excitatory synapse to long term effects on excitotoxic neuronal cell death. Thus, regulation has been shown to occur on multiple levels, including DNA transcription and protein translation, as well as posttranslational modification, which, in turn, may affect glutamate transporter protein targeting, localization and transport activity. DNA transcription and protein expression are more likely to play a role in chronic glutamate transporter regulation, such as the one needed for the triggering of glutamate excitotoxicity, as these events require more time to induce changes in glutamate uptake activity (for detailed reviews see Sattler and Rothstein 2006; Kim et al. 2011). On the other hand, posttranslational modifications, transporter trafficking or direct transporter protein modulation can occur very acutely within minutes and therefore respond to fast synaptic event requirements (for detailed review see Robinson 2006; Sattler and Rothstein 2006).
Early in vitro studies showed that cultured astrocytes require the presence of neuronal soluble factors to express glutamate transporter EAAT2/GLT-1, and to a lesser degree EAAT1/GLAST (Drejer et al. 1983; Gegelashvili et al. 1997; Swanson et al. 1997; Schlag et al. 1998).The search for these soluble factors began, and the cloning of the glutamate transporter promoter regions allowed for the identification of more defined pathways regulating transporter expression while confirming earlier findings. For example dibutyryl- cAMP (db-cAMP), epidermal growth factor (EGF) as well as transforming growth factor-α (TGF-α), κB-motif binding phosphor protein or pituitary adenylate cyclase-activating polypeptide (PACAP) have all been shown to increase EAAT2/GLT-1 expression (Eng et al. 1997; Swanson et al. 1997; Schlag et al. 1998; Figiel and Engele 2000; Zelenaia and Robinson 2000; Yang et al. 2009). Inhibitors of phosphatidylinositol 3-kinase (PI3K) and nuclear transcription factor κB (NF-κB) blocked the effects of EGF and db-cAMP, and cloning of the promoter regions confirmed the presence of regulatory transcription factor binding sites including NF-κB, N-myc and nuclear factor of activated T cells (NFAT) for EAAT2/GLT1 and NF-κB, cAMP responsive element binding protein (CREB), activating protein 1 (AP1) and GC-box elements for EAAT1/GLAST (Kim et al. 2003; Su et al. 2003; Li et al. 2006). These new studies now confirmed a transcriptional activation by measuring increased mRNA levels of EAAT2/GLT-1 and EAAT1/GLAST (Kim et al. 2003; Su et al. 2003; Li et al. 2006) and supported the idea that glutamate transporter regulation differs between transporter subtypes but can also differ between activation and repression of one individual factor (Sitcheran et al. 2005), emphasizing the complexity of the glutamate uptake system.
In addition to transcriptional regulation, Lin and colleagues also found evidence for translational regulation of 5ʹ-untranslated regions (UTRs) of EAAT2/GLT-1, both in vitro and in vivo (Tian et al. 2007). These regions responded to exposure of corticosterone and retinol with increased glutamate uptake activity without increased mRNA levels of EAAT2/GLT-1, suggesting that aside from transcriptional activation, translational activation of glutamate transporters offers another avenue to regulate synaptic glutamate homeostasis. The idea of translational activation was further supported by a recent study implicating a role of neuronal micro RNA miR-124a in increasing EAAT2/GLT-1 protein expression levels, but not mRNA levels (Morel et al. 2013).
Other studies suggested a role of epigenetic regulation of glutamate transporters via DNA methylation (Zschocke et al. 2007; Yang et al. 2010). In human glioma cell lines, the EAAT2/GLT-1 promoter shows hypermethylation, which was suggested to be at least partially responsible for the decreased expression of EAAT2/GLT-1 in glioma cells (Zschocke et al. 2007). Similar results were found in ALS postmortem patient tissue samples, were low levels of methylation were found, concomitant with low levels of EAAT2/GLT-1 expression (Yang et al. 2010). On the other hand, neuron-dependent increase in EAAT2/GLT-1 expression in cultured primary mouse astrocytes was paralleled with decreased methylation of CpG sites of the EAAT2/GLT-1 promoter, suggesting that neuron-dependent epigenetic regulation of EAAT2/GLT-1 is different from disease-mediated dysregulation of transporter expression, which seems to be independent of EAAT2/GLT-1 promoter methylation (Yang et al. 2010).
4.2.4 Dysregulation
Given the importance of glutamate transporter function in the maintenance of extracellular glutamate homeostasis and consequently prevention of neuronal cell death via glutamate excitotoxicity, loss of glutamate transporter activity has been studied in a number of neurodegenerative diseases with the hope of the development of therapeutic interventions to prevent neurodegeneration by glutamate toxicity. Particular interest was given to astroglial transporter EAAT2/GLT-1 due to its high prevalence in the CNS and major contribution to overall glutamate removal.
Mechanisms leading to the loss of transporter protein are still unknown, but can be caused on both, the transcriptional level as well as the translational level of protein synthesis. Loss of EAAT2/GLT-1 has been reported in acute and chronic neurodegenerative disorders , as well as psychiatric diseases and addiction (For review see Kim et al. 2011; Lin et al. 2012): ischemia/hypoxia (Rothstein et al. 1996; Martin et al. 1997; Inage et al. 1998; Fukamachi et al. 2001; Rao et al. 2001; Chen et al. 2005; Yeh et al. 2005) ; Huntington’s disease (HD) (Lievens et al. 2001; Behrens et al. 2002); Parkinson’s disease (PD) (Ginsberg et al. 1995; Levy et al. 1995), epilepsy (Bjornsen et al. 2007; Sarac et al. 2009; Kong et al. 2012); Multiple Sclerosis (Mitosek-Szewczyk et al. 2008); Alzheimer’s disease (AD) (Masliah et al. 1996, 2000; Li et al. 1997) and amyotrophic lateral sclerosis (ALS) (Rothstein et al. 1992; Bruijn et al. 1997; Howland et al. 2002); depression (Sanacora et al. 2004; Mineur et al. 2007; Sattler and Rothstein 2007; Gomez-Galan et al. 2013); alcohol dependence (Rao and Sari 2012); and cocaine addiction (Fischer et al. 2013). Most of these diseases/conditions report a loss of glutamate transporter protein and mRNA, suggesting a dysregulation on a transcriptional level, possibly due to lack of specific transcription factor activation. No detailed mechanisms have been elucidated yet to explain this phenomenon. Some disorders show loss of glutamate transport function without a change in transporter mRNA, indicating dysfunctional translational regulation during disease progression. For example, animal models for AD had no changes in EAAT2/GLT-1 mRNA levels, while glutamate uptake and transporter protein levels were significantly reduced (Masliah et al. 2000). Similar observations were made in postmortem tissue of frontal cortex of AD patients (Li et al. 1997). The mechanisms underlying reduced translational activity are not yet fully understood either, but a possible explanation could be inhibition of translation via highly structured cis elements in the 5ʹUTR sequence (Tian et al. 2007).
Another possible cause for decreased glutamate uptake is abnormal splicing of EAAT2/GLT-1 mRNA during disease progression (Lin et al. 1996; Maragakis et al. 2004; Sullivan et al. 2004; Lee and Pow 2010). The altered splice product could lead to the production of truncated EAAT2/GLT-1 protein or lead to mistargeting of the transporter, which could then be responsible for reduced glutamate transport activity. The hypothesis of a role of alternate splicing in glutamate transporter dysfunction has been supported recently by the idea of a high prevalence of RNA toxicity and subsequent altered RNA metabolism due to sequestration of RNA binding proteins in ALS, but also other neurodegenerative disorders (Belzil et al. 2012).
4.2.5 Therapeutic Potential
Given the long list of neurologic disorders showing decreased glutamate transporter function, it becomes obvious that increasing glutamate transporter activity offers an interesting therapeutic target that could be applicable for multiple neurodegenerative disease. The hypothesis is that restoring functional glutamate uptake will prevent accumulation of toxic concentrations of extracellular glutamate and consequently protect against excitotoxic neuronal cell death. Two early studies support this hypothesis using transgenic mice overexpressing GLT-1 (1.5–5 fold over baseline levels) (Sutherland et al. 2001; Guo et al. 2003). Crossing these mice with mutant superoxide dismutase 1 (SOD1) mice, an animal model for ALS, resulted in delayed onset of motor neuron degeneration and increased survival, suggesting that preventing loss of glutamate uptake protects against neurodegeneration . Similar findings were made recently, when an EAAT2 overexpressing mouse was tested in pilocarpine-induced status epilepticus (SE), resulting in reduced epileptogenic symptoms, including chronic seizure frequency and post-SE mortality rate (Kong et al. 2012). These studies strongly suggest that enhancing EAAT2/GLT-1 protein expression and function is a promising therapeutic approach.
Searching for pharmacological drug-like compounds enhancing EAAT2/GLT-1 function, Rothstein and colleagues identified that a group of US Food and Drug Administration approved beta lactam antibiotics increased glutamate transporter protein levels and function both in vitro and in vivo (Rothstein et al. 2005). Ceftriaxone, the beta lactam with the highest blood brain barrier penetration, was further characterized in the SOD1 mutant mouse model of ALS, resulting in increased protein levels of GLT-1, followed by increased survival, similar to what had been shown in the genetically altered mice (Rothstein et al. 2005). Since the initial discovery of ceftriaxone as a glutamate transporter enhancer and neuroprotectant, numerous animal models of neurodegenerative and psychiatric diseases , including Huntington’s Disease , Parkinson’s Disease , stroke , depression have been tested for ceftriaxone and reported neuroprotective activity concomitant with increased glutamate transporter expression and function (Lipski et al. 2007; Hota et al. 2008; Miller et al. 2008; Thone-Reineke et al. 2008; Ramos et al. 2010; Lai et al. 2011; Sondheimer and Knackstedt 2011). Furthermore, ceftriaxone has been tested in a National Institutes of Health-sponsored clinical trial resulting in a successful first and second phase of a multi-phase trial, but failing in the final 3rd phase due to lack of significant survival over the placebo group (Berry et al. 2013). In addition to ceftriaxone, we also identified harmine and thiamphenicol as EAAT2/GLT-1 transcriptional activator (Li et al. 2010; Sattler et al. 2011, 2013). From a drug discovery perspective, these compounds offer great opportunities for advancement towards lead compounds for future structure-activity relationship studies to improve potency as well as selectivity and drug delivery, as ceftriaxone e.g. cannot be administered orally.
While ceftriaxone was discovered as a transcriptional activator of EAAT2/GLT-1 (Lee et al. 2008), Lin and colleagues (Colton et al. 2010) were screening small molecule libraries for translational activators of EAAT2/GLT-1. Using a cell based enzyme-linked immunosorbent assay, the authors identified a novel lead series of active compounds, which are currently tested for in vivo efficacy in chronic and acute models of excitotoxicity (Lin et al. 2012).
Not many compounds have been identified that alter glutamate transporter function directly, by binding to the transporter protein. Parawixin 1, a spider toxin, was shown to increase EAAT2/GLT-1-mediated glutamate transport into the cells, not out of the cells, supposedly by speeding up the turnover of the glutamate transport cycle via conformational protein changes (Fontana et al. 2007; Torres-Salazar and Fahlke 2007). Drug discovery efforts on advancing this toxin towards the clinic are slowed down due to the lack of knowledge of the chemical structure of this protein.
4.2.6 Biomarker Potential
Reiterating the importance of a functional glutamate homeostasis it seems obvious, that opportunities to monitor functional glutamate uptake or the presence of glutamate transporters in the brain could present a valuable biomarker for both, disease detection as well as an evaluation of therapeutic efficacy during treatment in many neurological diseases , as suggested above. To our knowledge, our laboratory in collaboration with others has made the first progress on the development of two different biomarkers around the EAAT2/GLT-1 glutamate transporter protein.
The development of CNS biomarkers has been difficult partly due to the lack of availability of relevant tissue samples, i.e. live brain tissue. To overcome this challenge, we employed neural olfactory epithelial tissue to validate drug activity on astroglial proteins, including EAAT2/GLT-1. We validated this approach using the EAAT2/GLT-1 enhancing drug thiamphenicol, which was originally discovered together with ceftriaxone. We validated the effects of thiamphenicol on EAAT2/GLT-1 expression in olfactory epithelial tissue in a per-clinical rodent study as well as a phase 1 human trial, confirming biological activity of thiamphenicol at the drug target EAAT2/GLT-1 (Sattler et al. 2011).
In addition to the nasal biopsy approach, together with our collaborators from the University of Montana, we have been working on the development of an EAAT2/GLT-1 Positron Emission Tomography (PET) ligand biomarker . This PET ligand would allow us to visualize and quantify the levels of glutamate transporter protein in the CNS and spinal cord of patients. Ongoing studies have confirmed the specificity of this tracer in rodents and monkeys, moving this PET ligand towards first a first human safety trial by the beginning of 2014 (Gerdes and Sattler unpublished observation).
4.3 Monocarboxylate Transporters
The SLC16 gene family of monocarboxylate transporters (MCTs) consists of 14 members implicated in the transmembrane transport of short-chain metabolites. Out of these 14, four of them are known to be involved in the proton-coupled transport of monocarboxylates like pyruvate, L-lactate and ketone bodies across plasma membranes. They are classified according to their functional characterization as MCT1, MCT2, MCT3 and MCT4. Alternatively, they have also been classified as SLCA1, SLCA7, SLCA8 and SLCA3, respecively. We will use the former nomenclature throughout the remainder of the text. Other MCTs like MCT8 are known to be involved in thyroid hormone signaling (Friesema et al. 2003) whereas MCT10 is involved in the transmembrane transport of aromatic amino acids (Halestrap 2013). Unlike the ‘true’ monocarboxylate transporters, the transport of these substrates is not proton linked. The 8 other members of the SLC16 family have not been fully characterized so far and their implication under normal physiological situations or in disease still needs to be elucidated. Of all 14 monocarboxylates, only MCT1-MCT4 are known to be expressed in the CNS, with MCT1 and MCT4 mainly expressed in the glial cells, MCT2 in neurons and MCT3 specifically in the basolateral membrane of the retinal pigment epithelial cells (see below) .
4.3.1 Structure
MCTs have 12 transmembrane helices as based on hydropathy blots. Both the N-terminal as well as the C-terminal end is intracellularly located and there is a large cytoplasmic loop between helices 6 and 7 (Halestrap 2013). It is suggested that MCTs are never glycolysated, unlike their ancillary protein (see below). Most of our knowledge of MCTs comes from studying the MCT1 isoform and it is thought that this structure is commonly shared by all MCTs. Using molecular modeling in combination with site directed mutagenesis, Halestrap and colleagues have suggested that MCT1 changes its conformation from an ‘open’ state, during which extracellularly one hydrogen ion binds to the transporter followed by the binding of the monocarboxylate L-lactate, to a ‘closed’ state during which MCT1 undergoes a reorganization of the N- and C-terminal 6-helix halves and lactate acid can diffuse intracellularly (Galic et al. 2003; Manoharan et al. 2006; Wilson et al. 2009). Mutations of specific residues within the MCT1 structure might lead to changes in substrate specificity. To function properly, MCTs need an ancillary protein as a chaperone, which enables MCTs to be expressed at the cell surface. Generally, MCT1, MCT2 and MCT3 use basigin as their ancillary protein, while MCT2 uses embigin (Wilson et al. 2005). Without these chaperones, MCT1 and MCT4 locate to the perinuclear area and are not expressed at the plasma membrane (Kirk et al. 2000; Wilson et al. 2005). Basigin/embigin have a single transmembrane domain and a very short intracellular C-terminal region (Iacono et al. 2007). They are highly glycosylated, unlike MCTs (Iacono et al. 2007). Through the glycosylated domains of their ancillary proteins, MCT expression might become more susceptible to expression regulation as would be predicted solely based on its own structure.
4.3.2 Expression
In mammals MCTs are widely expressed throughout different tissues. MCT1 is ubiquitously expressed with exception of the beta-cells of the endocrine pancreas (Halestrap 2013). MCT2 is widely expressed, highly in testis, and less so in spleen, heart, kidney, skeletal muscle and in the CNS (Garcia et al. 1995; Jackson et al. 1997; Halestrap 2013). MCT3 is exclusively expressed on the basolateral surface of the retinal pigment epithelial cells, regulating retinal osmotic pressure (Philp et al. 1995). MCT4 is mainly expressed in highly glycolytically active tissue, e.g., neonatal heart, chondrocytes and skeletal muscle (Hatta et al. 2001; Halestrap 2013). Other characterized monocarboxylate transporters like the thyroid hormone transporter MCT8 is also expressed ubiquitously (Visser et al. 2011), whereas the aromatic amino acid transporter MCT10 is mainly expressed in kidney, intestine and heart (Halestrap 2013). As mentioned earlier, In the CNS, four MCT isoforms have been identified; MCT1, MCT2, MCT3 and MCT4. MCT1, previously thought to be chiefly expressed in astrocytes and endothelial cells, is mainly expressed in oligodendroglial cells (Rinholm et al. 2011; Lee et al. 2012), the cells that myelinate axons to enable fast conduction of electric pulses along neurons. MCT1 is also expressed in some specific neuronal subpopulations, e.g. Purkinje neurons in the cerebellum (Lee et al. 2012). MCT4 is mainly expressed by astrocytes (Rafiki et al. 2003), the cells that are involved in a wide range of functions ranging from ion and energy homeostasis to glutamate uptake from the synaptic cleft (see below). MCT2 is mainly expressed by neurons (Pierre et al. 2000), especially in the mitochondrial enriched post-synaptic density, where it might be involved in trafficking of AMPA glutamate receptors (Bergersen et al. 2005; Maekawa et al. 2009), more specifically the sorting of their GluA2 subunits which absence determine AMPA channel Ca2+ permeability (Hollmann et al. 1991). It has to be mentioned here that for the localization of MCT2 and MCT4, the majority of the findings are based on antibody based immunodetection solely and have to be taken with caution (Pierre et al. 2000; Rafiki et al. 2003).
4.3.3 Physiology
In the CNS MCTs transport monocarboxylates bidirectionally, depending on the relative concentration of lactate and hydrogen ions across plasma membranes. MCTs can function as an exporter or importer. A net cellular flux is determined by the relative contribution of import versus export. Of the different MCTs expressed in the CNS, MCT2 has the highest affinity for its substrates (Km for L-lactate 0.74 mM), followed by MCT1 (Km for lactate 4.5 mM) and MCT4 (Km for lactate 28 mM) (Halestrap and Price 1999; Halestrap 2012). Compared to the other MCTs, MCT4 has an extremely low affinity for substrates, making it more suitable for lactate export rather than import in highly glycolytic cells which produce large amounts of lactate. As this accumulation of lactate leads to a reduction of the intracellular pH, cells need to export lactate with hydrogen ions to protect them from acidosis. The MCT4 physiological characteristics make it ideal for this purpose. With its high Km for lactate, it is only saturated at higher lactate concentrations. In addition, MCT4 has a high Km for pyruvate, preventing pyruvate from being transported to the extracellular space. Pyruvate export would lead to a drop in intracellular NAD+ levels and an impairment in maintaining glycolysis. In addition, intracellular pyruvate to lactate conversion restores the NAD+ levels and enables glycolysis to continue. The low Km values for lactate for MCT1 and MCT2 make them more suitable for lactate import rather than export and therefore allow the cells to use lactate for subsequent oxidation, lipogenesis or gluconeogenesis. Consequently, these transporters are found in cells whose metabolism relies on oxidative metabolism rather than glycolysis, e.g., in the adult heart and in neurons. MCT3’s affinity for lactate is probably low, but is not well characterized. The specific physiological characteristics of the different MCT isoforms could allow for the discussion of subtype specific inhibitors which in turn could provide a great tool for a wide range of MCT research (see below).
Importantly, to date, most MCT inhibitors still lack specificity. An example is α-cyano-4-hydroxycinnamate (CHC), which blocks MCTs but is twice as potent in blocking the mitochondrial pyruvate transporter (Halestrap 2013). Similarly, the widely used MCT inhibitors 4,4’-di-isothiocyanostilbene-2,2’-disulphonate (DIDS) and 4,4’-dibenzamidostilbene-2,2’-disulphonate (DBDS) inhibit the chloride/bicarbonate transporter far more potently than MCTs (Poole and Halestrap 1991; Halestrap 2013; Kucherenko et al. 2013). Therefore their use has to be interpreted with care. More recently, MCT inhibitor AR-C155858 was discovered with a very low Ki of around 2 nM, making it a very potent MCT blocker (Ovens et al. 2010). It inhibits both MCT1 and MCT2 but not MCT4 and has shown great therapeutic potential as an immunosuppressant (see below) (Ovens et al. 2010).
As mentioned earlier, different tissues express different MCT subtypes and cells express specific MCTs based on their physiological characteristics, i.e. whether they are more glycolytically active and produce lactate or whether they rather take up lactate from the extracellular space for oxidation, lipogenesis or gluconeogenesis.
In the CNS, some cell types are more glycolytically active while other cells are more oxidative. The most notable example is how glycolytically active, lactate producing astrocytes are metabolically coupled to neurons to sustain the latter’s increased metabolic needs during neuronal activation, the so-called neuron-astrocyte lactate shuttle, as proposed by Magistretti and colleagues (Pellerin and Magistretti 1994). In fact not only are neurons unable to increase their glycolytic rate (Herrero-Mendez et al. 2009), they are also unable to store glucose as glycogen as their glycogen synthase enzyme is rendered inactive (Vilchez et al. 2007), and so might need other means to sustain their metabolism , especially under conditions of increased demand. Astrocytes are well known to upregulate glycolysis in situations of increased metabolic needs. As described above, astrocytes take up glutamate via glutamate transporters . This transport is coupled to three sodium ions, which activates the Na+/K+ pump in the astrocytic cell membrane in order to maintain its membrane potential. The Na+/K+ pump is ATP dependent, leading to increased glucose uptake through glucose transporters or increased glycogenolysis from their intracellular glycogen stores. Glucose is then converted to lactate to produce sufficient ATP levels. As astrocytes pile up lactate rapidly, they use the high lactate capacity MCT4 transporter to export lactate (Rafiki et al. 2003). Lactate could be taken up by neurons through MCT2 and converted back to pyruvate and used to sustain their own metabolism (Pierre et al. 2000). This enhancement of glucose uptake in response to glutamate uptake is now widely recognized (Takahashi et al. 1995; Keller et al. 1996; Bittner et al. 2010). The neuron-astrocyte lactate shuttle hypothesis does not rule out the role of glucose oxidation in maintaining neuronal metabolism (neurons express their own glucose transporter, Glut3 (Maher et al. 1991)), but lactate seems to be preferred over glucose, especially under conditions of increased demand, e.g., during neuronal activation, as has been shown both in vitro (Bouzier-Sore et al. 2003; Itoh et al. 2003; Bouzier-Sore et al. 2006; Ivanov et al. 2011) as well as in vivo (Smith et al. 2003; Serres et al. 2004; Wyss et al. 2011). Even more, lactate seems to have more neurotrophic potential than glucose in models of neuroinjury, as lactate, but not equicaloric glucose, is able to reduce stroke volume in an animal model of hypoxia (Schurr et al. 1997).
Recently Rothstein and colleagues have shaken up the astrocyte neuron lactate shuttle paradigm considerably as they have shown that another cell type in the CNS, the oligodendrocyte , is also involved in the metabolic coupling in the CNS. Unlike previous reports about MCT1 expression in the brain as mainly being detected in astrocytes and endothelial cells, they found using a bacterial artificial chromosome (BAC) transgenic mouse that it is the oligodendrocyte rather than any other cell type who expresses MCT1 in vivo (Lee et al. 2012). In organotypic spinal cord slice cultures, lactate released through oligodendrocyte MCT1 sustains neuronal energy demands. Blocking oligodendrocyte MCT1 transporters leads to motor neuronal cell death, even while MCT4 expression in astrocytes was not affected. This neuronal cell death could be rescued by the addition of lactate to the culture medium. This data suggest that oligodendrocytes export lactate through MCT1 and provide this essential nutrient to neurons (Fig. 4.1b). Similar results were found in vivo (Lee et al. 2012). MCT1 heterozygous knockout mice, as well as mice injected with oligodendrocyte specific lentiviral knockdown of MCT1, showed signs of neuronal degeneration in the absence of overt oligodendrocyte pathology, indicating that a metabolic flux through MCT1 expressed by oligodendrocytes is an essential contributor to neuronal viability (Lee et al. 2012). One cannot rule out however that astrocytes themselves are also able to provide neurons to some extent with lactate directly through MCT4, as suggested by the astrocyte-neuron lactate shuttle hypothesis. On the other hand astrocytes, being able to enhance its own glycolytic rate, could provide oligodendrocytes with lactate through astrocyte-oligodendrocyte gap junctions (Fig. 4.1a). These gap junctions consist of various paired connexon (i.e., connexin hemichannel) isoforms formed by connexin-47 and connexin-32 expressed on oligodendrocytes and connexin-43 and connexin-30 expressed on astrocytes and enable exchange of metabolites between both cell types (Dermietzel et al. 1989; Nagy et al. 1999; Odermatt et al. 2003). After reaching the oligodendrocyte through these coupled hemichannels, lactate can be metabolized by oligodendrocytes to sustain their own metabolic needs or directly provided to neurons after export through MCT1. Another option is that oligodendrocytes produce lactate themselves through glycolysis after taking up glucose through gap junctions or from the extracellular space through their own glucose transporters. It has been shown that oligodendrocytes are able to maintain their myelin sheath and sustain axonal integrity in the absence of functional oxidative phosphorylation, when their function is dependent on glycolysis or connexin mediated import of astrocyte ATP (Funfschilling et al. 2012). It is not known at this point though, whether the lactate provided to neurons is being generated by astrocytes or oligodendrocytes . In fact it is not known whether oligodendrocytes are able to increase their glycolytic rate under conditions of increased energy demand just as astrocytes do. Therefore, it is suggested that astrocytes convert glucose to lactate first, after which lactate enters the oligodendrocytes through gap junctions and is then provided to neurons through MCT1 mediated export. This is further supported by the fact that astrocytes are able to enhance glycolysis and are efficiently coupled to blood vessels from where they take up glucose through glucose transporters (Nakazawa and Ishikawa 1998). Astrocytes also express the high capacity lactate transporter MCT4 to maintain its own viability when lactate levels rise high and express high levels of hexokinase, which converts glucose to glucose-6-phosphate in the first step of glycolysis (Rafiki et al. 2003). On the other hand, specific oligodendrocyte characteristics make these cells more suited to provide neurons with lactate . Unlike astrocytes, oligodendrocytes are very closely interacting with neurons all along their axons and can release metabolites to neurons through Schmidt-Lanterman incisures in their myelin sheath. Astrocytes, with their end feet touching blood vessels are more ideal for glucose uptake from the blood, transporting glucose along their astrocytic syncytium to regions with increased energy demand, and providing oligodendrocytes with lactate for those regions not covered by astrocyte processes.
Although the validity of the glial-neuron lactate shuttle is accepted by many researchers in the field, it has also been challenged by many other investigators, and is still controversial to some extent. One can argue, e.g., that some of the findings are based on extensive in vitro studies using neuron-glia co-cultures and might not be relevant for in vivo neuronal metabolism. This critique can be challenged by several recent in vivo experiments. For example, in an anesthetized rat model, it has been shown that neurons prefer lactate over glucose as an energetic source, as artificial increased blood lactate levels reduces glucose consumption under both normal conditions as well as during neuronal stimulation (Wyss et al. 2011). Using two-photon in vivo imaging of 6-deoxy-N-(7-nitrobenz-2-oxa-1,3-diazol-4-yl)-aminoglucose in the somatosensory cortex of the rat, it has been shown that under resting conditions glucose is being taken up by both neurons and astrocytes , but in response to whisker stimulation, astrocytes enhance their glucose uptake while neurons do not, which is in agreement with the glial-neuron lactate shuttle (Chuquet et al. 2010). Also, in humans lactate is important for brain metabolism, as high as 10 % under normal physiological conditions and increasingly so (up till 60 %) in conditions of increased demand (Boumezbeur et al. 2010).
Secondly, some studies insist that glucose is the major energy source for neurons, rather than lactate , and might be used by neurons directly without astrocyte interplay through neuronal Glut3 transporters (Simpson et al. 2007; Mangia et al. 2009; DiNuzzo et al. 2010). Although it is not disputed that glucose itself is able to sustain neuronal energy demands, especially under normal physiological conditions, the contribution of lactate cannot be disregarded. Firstly, glucose transporter Glut3 knockout mice develop normally and do not show any obvious phenotype, which would be in discrepancy with glucose as a direct energy substrate for neurons (Schmidt et al. 2008; Stuart et al. 2011). On the other hand, knockout of the astrocyte glucose transporter Glut1 in mice, leads to a strong phenotype with motor impairment and seizures , in accordance that glucose has to be converted to lactate first by astrocytes, before lactate can be used as an energy substrate by neurons (Wang et al. 2006) . In an elegant contextual learning study in mice, it was found that long-term memory was impaired when astrocytic MCT4 or MCT1 was knocked down in the hippocampus with antisense treatment (Suzuki et al. 2011). Interestingly, this impairment could be rescued by adding lactate but not equicaloric glucose to the mouse hippocampus. Even more, when the authors knocked down neuronal MCT2 expression, long term memory formation was also impaired which could not be rescued by adding lactate, suggesting that (1) astrocyte and their respective MCTs (MCT1 or MCT4) are essential in providing neurons with energy substrates and that (2) neurons use lactate, taken up by MCT2, rather than glucose, to maintain their long term memory formation. One cannot rule out however, that other metabolites like, e.g., ketone bodies might also be involved in maintaining the neuronal energy balance.
4.3.4 Regulation
Although there are some examples of short-term regulation of MCT expression, these are very rare. It has been suggested that MCT1 and MCT4 expression increases through the intracellular interaction with carbonic anhydrase 2 which increases transport activity, while MCT2 expression is enhanced through an extracellular interaction with carbonic anhydrase 4 (Klier et al. 2011). Most of what is known about MCT expression regulation are mechanisms involved in transcriptional and posttranscriptional regulation, which usually take long before exerting its effects. MCT1 expression in muscle is increased in response to enhanced physical activity and lactate transport is decreased in response to denervation (Pilegaard et al. 1998; Pilegaard et al. 1999). In the brain, MCT1 expression is upregulated in response to hyperglycemia and ketosis (Leino et al. 2001; Canis et al. 2009). Two mechanisms have been proposed that stimulate MCT1 expression, both through increased Ca2+ levels. One enhances calcineurin activity, which dephosphorylates and activates nuclear factor of activated T-cells (NFAT) which then regulates MCT1 expression levels by binding to NFAT consensus binding sites on the MCT1 promoter (Halestrap 2013). T-lymphocytes for example increase their MCT1 expression levels during proliferation to maintain their pH levels. Both Inhibition of calcineurin activity with cyclosporin A as well as a MCT1 inhibitor are efficient in preventing T-lymphocyte proliferation (Murray et al. 2005). MCT1 expression is also regulated through AMP activated protein kinase (AMPK) (Takimoto et al. 2013), which is modulated by 5-aminoimidazole-4-carboxamide-1-β-D-ribonucleoside (AICAR) expression levels (Halestrap 2013). It is not clear whether AICAR increases or decreases MCT1 levels, as opposing results have been reported previously (Galardo et al. 2007; Halestrap 2013). It has further been reported that MCT1 promoter activity is also regulated by protein kinase C (PKC) levels, mediated by transcription factor activator protein 2 (AP2) (Saksena et al. 2009). Interestingly, in the beta cells of the pancreas, MCT1 is not expressed (Otonkoski et al. 2007). One possibility is that tissue specific methylation of CpG islands in the MCT1 promoter prevent MCT1 expression (Mueller and von Deimling 2009). For example, it has recently been reported that the extent of methylation of CpG islands in the MCT4 promoter is correlated with reduced MCT4 expression and prolonged survival in patients with cancer related death (Fisel et al. 2013). A recent study suggested that the MCT1 promoter activity is not regulated by CpG methylation, but rather by miRNA mediated gene suppression. The authors identified miRNA 29 isoforms which were instrumental in suppressing MCT1 expression in the beta cells of the pancreas (Pullen et al. 2011). Individuals with distinct mutations in these regions display exercise induced hyperglycemia due to increased insulin secretion from the beta cells in response to elevated blood lactate levels (Otonkoski et al. 2007) Lastly, intracellular pools of MCT1 might increase MCT1 expression at the plasma membrane irrespective of transcriptional regulation, as occurs in hypertrophied heart tissue (Johannsson et al. 2001).
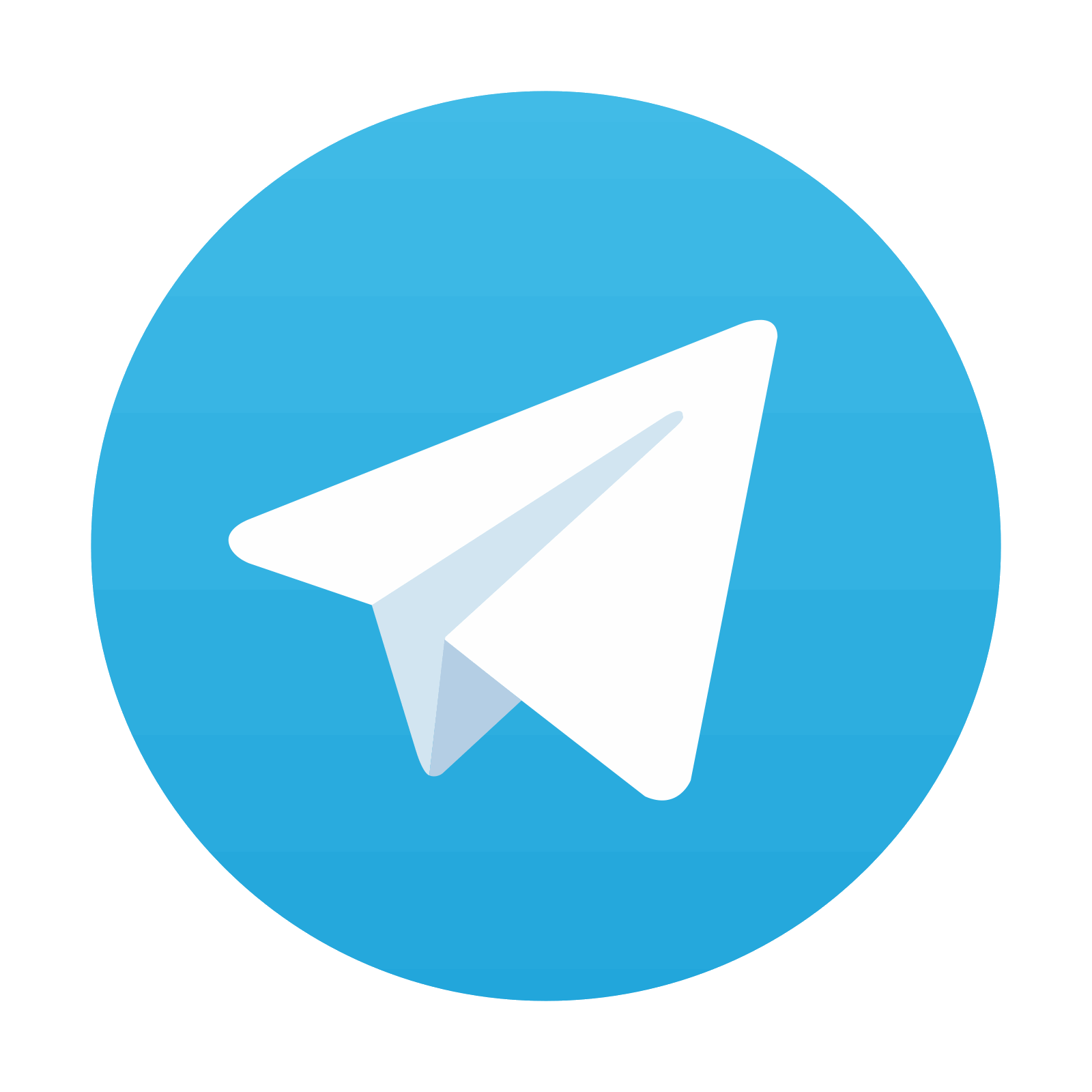
Stay updated, free articles. Join our Telegram channel
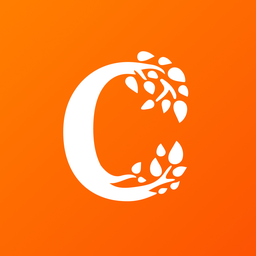
Full access? Get Clinical Tree
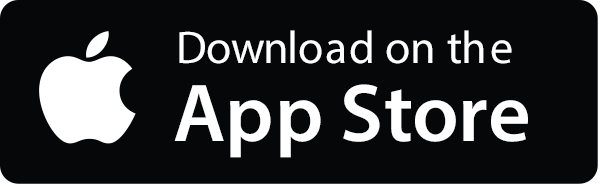
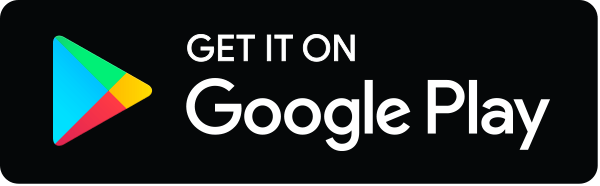