![]() FIGURE 4.2. Development of human heart. This diagram shows the formation of the four heart chambers. |
atrial appendage morphology. The normal left atrial appendage (LAA) is an elongated, hooked, tubular structure with crenulations and a small junction with the venous portion of the atrium. In contrast, the typical right atrial appendage (RAA) is triangular and broad with a broad connection to the venous inflow tract. On the right, a groove or crest demarcates the appendage from the atrial body; on the left, this is not present. If atrial appendage identity cannot be reliably established, another methodology for establishing situs is the relationship of the airways to the pulmonary arteries. Normally, the right upper lobe bronchus is eparterial, with a takeoff superior to the origin of the right pulmonary artery. In contrast, the left upper lobe bronchus is normally hyparterial, taking off below the origin of the left pulmonary artery. Complete mirror-image situs anatomy is known as situs inversus. Other variations are known as situs ambiguous or heterotaxy and are most closely associated with underlying CHD.1,2,3,4
and may be asymptomatic6 (Fig. 4.3). Pulmonary venous branching is highly variable. Three right pulmonary veins (upper, middle, and lower) or two left pulmonary veins with a common ostium are common variants. In addition, the ostium of the left lower pulmonary vein is often smaller than that of other pulmonary veins, as it drapes over the descending thoracic aorta.7
first examination to suggest an underlying cardiovascular malformation. A systematic approach helps to avoid overlooking potential interpretive clues. Postsurgical material, support devices, and abnormal calcifications are usually plainly evident. Situs abnormalities can be readily detected by scrutinizing the stomach, spleen, and liver position and tracheobronchial branching pattern. While complete mirrorimage situs (situs inversus) is associated with no greater prevalence of CHD compared to normal situs (situs solitus), rates of CHD are significantly greater with intermediate situs variations (situs ambiguous, heterotaxy). Similarly, an anomalous position of the great vessels may be visible and should raise suspicion for underlying CHD. For example, a (single) right aortic arch, which manifests on radiographs as a right aortic knob deviating the trachea leftward of its expected position (normally slightly rightward of midline), is associated with CHD in 90% of cases (Fig. 4.5). Finally, an abnormal position of the cardiac apex in a location other than the left hemithorax (levocardia), that is, in the right hemithorax (dextrocardia) or in the midline (mesocardia), warrants attention. Of note, the apex position is not dependent on the anatomic arrangement of intracardiac and visceral structures.12,13,14,15,16,17,18
to pulmonary venous dilatation and migration of fluid into the perivascular tissues; such findings may be seen, for example, with impaired myocardial contractility. Concomitant lung disease may obscure the pulmonary vasculature, while enlargement of pulmonary vessels or cardiac chambers may secondarily cause focal atelectasis or air trapping.12,13,14,15,16,17,18
TABLE 4.1 Sample CT Angiography Contrast Injection Protocols with Fixed Scan Delay | ||||||||||||||||||||||||||||||||||||||||||||||||||||||||||||||||||||||||||||||||||||||||||||||||||||||
---|---|---|---|---|---|---|---|---|---|---|---|---|---|---|---|---|---|---|---|---|---|---|---|---|---|---|---|---|---|---|---|---|---|---|---|---|---|---|---|---|---|---|---|---|---|---|---|---|---|---|---|---|---|---|---|---|---|---|---|---|---|---|---|---|---|---|---|---|---|---|---|---|---|---|---|---|---|---|---|---|---|---|---|---|---|---|---|---|---|---|---|---|---|---|---|---|---|---|---|---|---|---|
|
Extracardiac structures such as the lungs and airways are also optimally evaluated. Postprocessing software allows multiplanar reformatting, maximum and minimum intensity projection reconstructions, and 3D volume rendering, facilitating visualization of complex CHD for diagnostic and presurgical planning purposes. Electrocardiographic (ECG) gating may be used not only to minimize image degradation from cardiac motion (e.g., as may be needed for coronary imaging) but also to quantify ventricular and regurgitant volumes, ventricular function, myocardial mass, cardiac output, shunt flow, and pulmonary to systemic flow ratios (Qp:Qs).12,14,15,21,22,23,24,25,26
TABLE 4.2 CT Angiography Scan Considerations by Indication | |||||||||||||||||||||||||||||||||||||||||||||
---|---|---|---|---|---|---|---|---|---|---|---|---|---|---|---|---|---|---|---|---|---|---|---|---|---|---|---|---|---|---|---|---|---|---|---|---|---|---|---|---|---|---|---|---|---|
|
TABLE 4.3 Representative Thoracic CT Angiography Scan Parameters | ||||||||||||||||||||||||
---|---|---|---|---|---|---|---|---|---|---|---|---|---|---|---|---|---|---|---|---|---|---|---|---|
|
required, MRI is generally preferred to CT, unless patients are too ill to tolerate its longer scan times or it is contraindicated due to incompatible devices.12,21
TABLE 4.4 Representative CMR Protocol for Structural Heart Disease | ||||||||||||||||
---|---|---|---|---|---|---|---|---|---|---|---|---|---|---|---|---|
|
TABLE 4.5 Representative CMR Protocol for Congenital Heart Disease (Repaired Tetralogy of Fallot) | ||||||||||||||
---|---|---|---|---|---|---|---|---|---|---|---|---|---|---|
|
requiring long reconstruction times and specialized software; however, these shortcomings are being addressed.12,21,40,41,42,43,44,45,46,47,48,49,50,51 The addition of ultrashort echo time (UTE) MR sequences can also allow simultaneous assessment of the airways and lungs, if indicated.51
was 0.76 mSv for children under one year of age compared to 13.4 mSv for catheterization.23 Thus, it is not surprising that many institutions reserve catheterization for situations in which highly accurate hemodynamic data are crucial or interventional procedures are anticipated.
![]() FIGURE 4.7. A 3-year-old boy with an atrial septal defect. Frontal chest radiograph shows increased pulmonary vascularity and cardiac enlargement. |
atrial septum is actually intact, but the border between the SVC and some of the right pulmonary veins is not, allowing pulmonary venous blood to pass directly into the SVC and then the RA. The atria then communicate at the pulmonary vein confluence.56,66,67 Sinus venosus ASD is associated with right upper lobe partial anomalous venous return and persistent left SVC (Fig. 4.13B).
![]() FIGURE 4.11. A 5-year-old boy with ostium secundum atrial septal defect (ASD). Frontal chest radiograph shows increased pulmonary vascularity and cardiomegaly. |
the LA to the coronary sinus and then the RA. In turn, the coronary sinus becomes widened. This defect is typically associated with a left SVC and, in some cases, total anomalous pulmonary venous return (TAPVR) or heterotaxy syndromes.56,68,69
![]() FIGURE 4.15. A 6-year-old girl with ventricular septal defect (VSD). Frontal chest radiograph shows increased pulmonary vascularity and cardiac enlargement. |
bow into the VSD, leading to AR. This defect comprises only 5% to 8% of VSDs in Western countries but up to 30% in Asia.27,28,56,77
almost always necessary and is performed between the 3rd and 6th months of life for complete defects and between 2 and 4 years of age for partial and intermediate defects. Once irreversible pulmonary hypertension has occurred, however, surgery is contraindicated.31,32,56
However, therapy for Eisenmenger syndrome is generally palliative. The unfortunately poor prognosis of this syndrome highlights the importance of early detection, monitoring, and treatment of CHD for prevention of future complications.33,34,35,56
![]() FIGURE 4.22. A 9-year-old boy with tetralogy of Fallot with pulmonary atresia. Axial enhanced CT image shows pulmonary atresia (arrow). Note the mediastinal collaterals. RA, right aortic arch. |
of cyanosis; with only mild RVOT obstruction, neonates may be not cyanotic (“pink tetralogy”). The absent pulmonary valve form of TOF is unique in that the markedly enlarged PAs cause tracheobronchial compression and resultant respiratory distress.12,36,37,56,78,79,80,81,82,83,84,85,86,87
![]() FIGURE 4.23. A 3-year-old with tetralogy of Fallot with absent pulmonary valve. Axial enhanced CT image shows dilated right (RPA) and left (LPA) pulmonary arteries. |
artery valved conduit (usually a homograft). In pediatric patients with significant symptoms or cyanosis, a modified Blalock-Taussig (BT) shunt, typically consisting of a Gore-Tex graft from the right or left subclavian artery to the ipsilateral pulmonary artery, may be used to augment pulmonary blood flow. Other palliative procedures used as bridges to definitive surgery may include balloon pulmonary valvuloplasty or placement of an RVOT stent. In TOF with pulmonary atresia, initial maintenance of ductal flow is critical and may be accomplished via infusion of prostaglandin E1 (PGE1) and, sometimes, ductal stenting. Treatment in the presence of MAPCAs is complex but, in general, consists of multistage surgeries and catheterization to augment the PAs and allow forward pulmonary blood flow; the use of MAPCAs to supplement the native PAs is known as “unifocalization.” In TOF with absent pulmonary valve, management includes partial resection and repair of the aneurysmal PAs. After definitive repair, pediatric patients with TOF are at risk for complications ranging from RV dysfunction to arrhythmia to sudden death and require long-term monitoring, for instance, to determine the timing of PVR, as previously described.12,36,37,56,78,79,80,81,82,83,84,85,86,87
Overall, TGA is rare, with an incidence of 1 in 2,000 to 5,000 live births. Nonetheless, TGA comprises 5% to 7% of all CHD and 10% of all neonatal cyanotic CHD. TGA has a male predominance of 1.5-3:1 and arises most commonly in infants of diabetic mothers. Other risk factors include fetal exposure to antiepileptic drugs and herbicides.12,37,56,78,96,97
baffles, as well as optimal visualization of common sites of complications after surgery. MRI is also most valuable for postoperative assessment, both providing anatomic evaluation and allowing accurate quantification of biventricular function and assessment of maximum velocity at any sites of obstruction for pressure gradient estimation. The role of cardiac catheterization for TGA diagnosis has declined, except for coronary artery assessment, depending on local cardiac CT expertise. However, palliative procedures such as balloon atrial septostomy can be performed at the time of catheterization.12,37,56,78,96,97
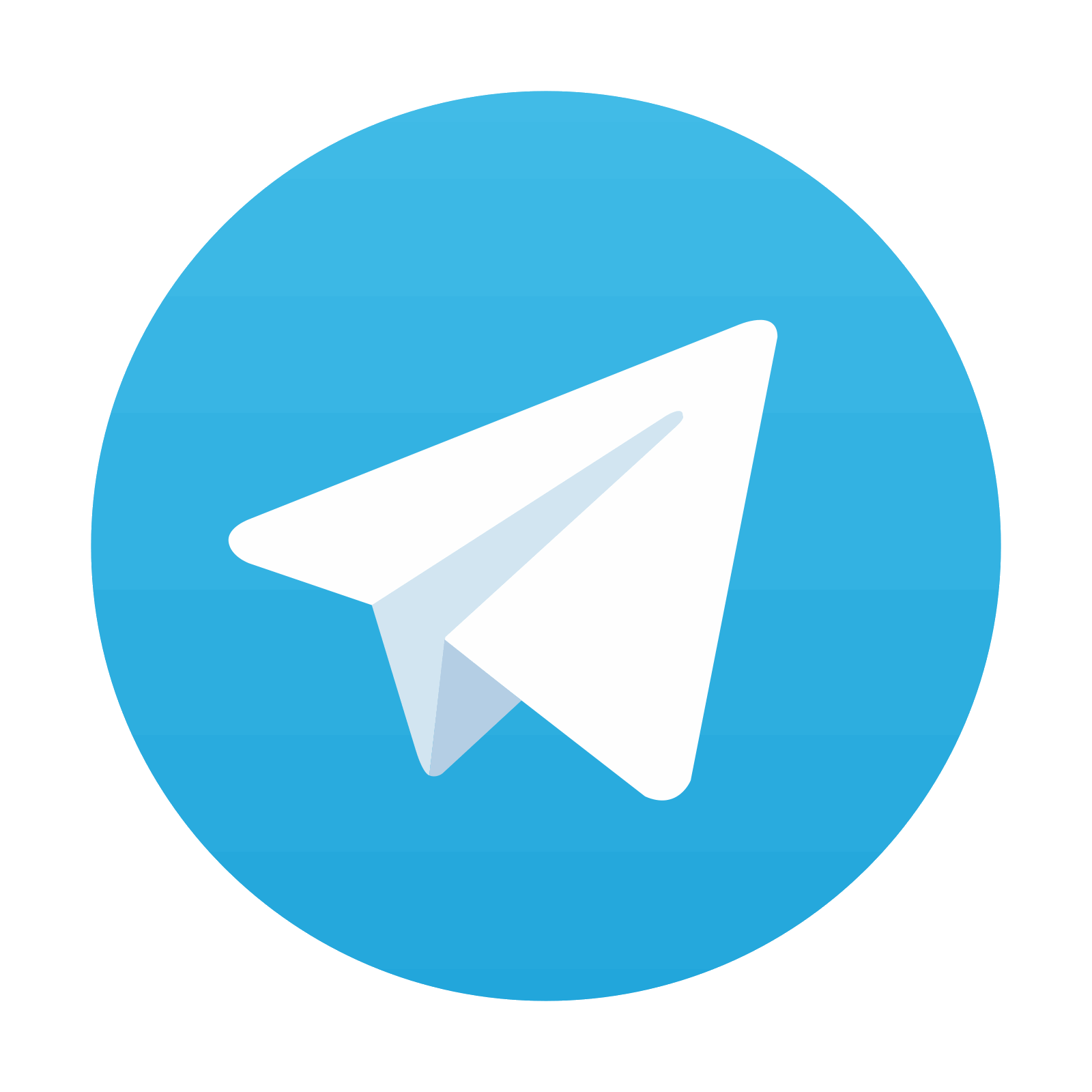
Stay updated, free articles. Join our Telegram channel
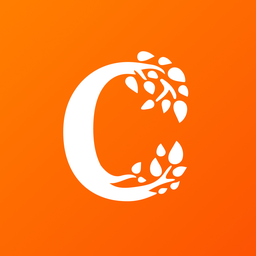
Full access? Get Clinical Tree
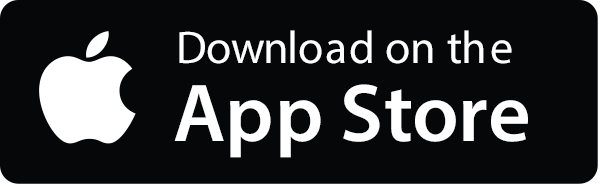
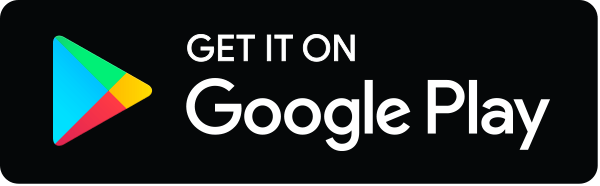
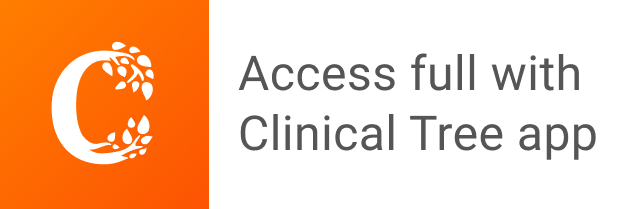