Fig. 2.1
(a) Number of citations per year indexed by Medline from 1966 to 2005 under subject headings related to important non-infectious, neuropsychiatric, infectious and sexually transmitted diseases. (b) Number of citations in the same disease categories additionally cross-referenced with subject headings related to social factors. From Cohen et al. (2007)
The shift to social epidemiology and the work on socio-economic gradients of health, the role of social networks or the influence of built environments—to name but a few topics—represented a move away from “focusing on clinical diseases, one at a time” to the consideration of “psychosocial and cultural forces that compromise a person’s ability to withstand insult” and establishing “the link between social forces and biologic processes” (Leonard Syme in Boyce 2011). The hope for preventive medicine represented the same “translational” aspiration of social epidemiology/medicine as that of the “hygienic” movement at the turn of the previous century.
In parallel with the emergence of social epidemiology, other strong trends were taking shape: molecular epidemiology (Kilbourne 1973; Schulte and Perera 1993) and genetic epidemiology (Morton et al. 1978; Khoury et al. 2004). The former returned primarily to the problem of infectious diseases, while the latter turned its attention to the study of genetic cases of non-infectious diseases, often the same ones studied by social epidemiologists. More recently, building on the successes of the Human Genome Project (HGP) (see Sect. 2.2), epidemiology joined forces with genomics to embark on “human genome epidemiology” (Khoury et al. 2004, 2010); the work discussed in the two editions of the Khoury et al. book is directly relevant to the topic of population neuroscience.
In summary, epidemiology traces its roots to the application of the “numerical method” (i.e. statistics) in the search for causes of epidemics and, in general, determinants of population health. From its initial interest in infectious diseases, it quickly expanded its scope to non-infectious diseases and, in the past 50 years, brought in tools and concepts from other disciplines, thus establishing new “hybrids”, such as social epidemiology and genetic epidemiology.
2.2 Genetics
In 1866, Gregor Mendel formulated the laws of inheritance and introduced the concept of the allele as a fundamental unit of heredity (Mendel 1886). He used the “numerical method” to derive statistical rules explaining the pattern of observations made during his experimental work on plant hybridization; this work was carried out in a garden of an Augustinian monastery (Brno, Moravia, present-day Czech Republic) between 1856 and 1865. Unfortunately, Mendel’s publication was missed by the mainstream science of the times, and his laws on inheritance were rediscovered only in 1900 by de Vries, Correns and von Tschermak. In the subsequent 50 years, a number of discoveries were made, laying down the biological foundations of genetics. Thus, in 1902, Walter Sutton suggested that “… the association of paternal and maternal chromosomes in pairs and the subsequent separation during the reducing division … may constitute the physical basis of the Mendelian law of heredity” (Sutton 1902, as quoted in Crow and Crow 2002).1 The subsequent work established that “genes” do indeed reside on chromosomes (1910), that chromosomes contain DNA (1933) and that genes code for proteins (1941). Then, DNA was purified (1944), its first picture taken with X-ray diffraction (1952), and its 3D structure, a double helix, solved by Watson and Crick (1953; Fig. 2.2).
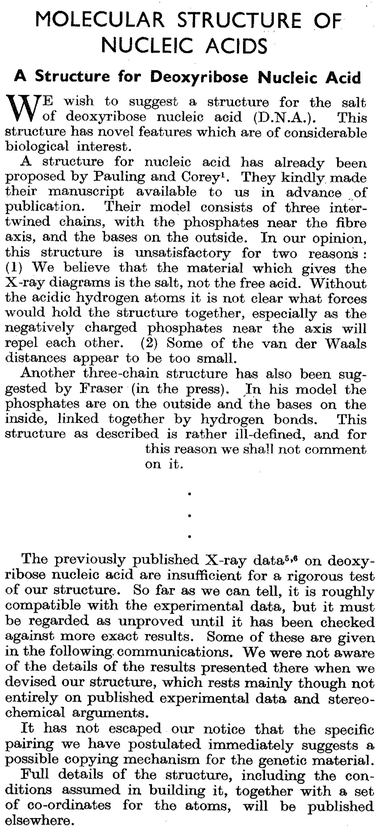
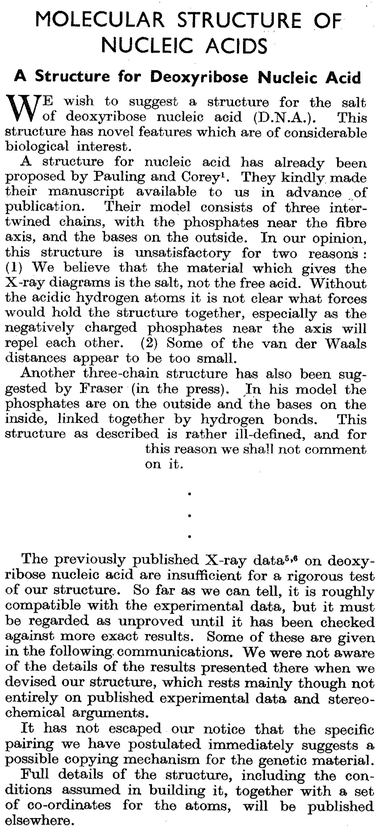
Fig. 2.2
Two excerpts from the one-page report by Watson and Crick on DNA structure. From Watson and Crick (1953)
The next 20 years saw, among other discoveries, the cracking of the genetic code (1966)2 and the isolation of restriction enzymes, a key discovery allowing the “cutting and pasting” of DNA. The following 20 years introduced two key methodological advancements, namely an electrophoresis-based method for DNA sequencing (1977) and polymerase chain reaction (1983) that enabled most of the work carried out in the 1990s by the HGP. The first disease-causing gene was identified (1989; cystic fibrosis trans-membrane conductance regulator), and the idea of doing genetic “fingerprinting” based on DNA polymorphisms was put forward (1989).
In 1990, the U.S. National Institutes of Health (NIH) and Department of Energy (DOE), together with their international partners, launched the HGP. Over the next 15 years, and with an annual budget of $ 200 million provided by the NIH and DOE, the HGP’s plan was to map and sequence the 3.2-billion-nucleotide-long genome: creating genetic and physical maps of the human genome, identifying all genes and sequencing the entire DNA (Watson and Jordan 1989). The initial plan for the genetic map envisaged one containing ~1,000 highly informative markers, so-called microsatellites, placed—on average—with an interval of ~2–5 million nucleotides. The physical location of these markers, and genes, had to be specified in real “mile stones” placed on the genome’s road: The plan called for the creation of a physical map to be based on the sequence-tagged sites3; a total of 30,000 of such sites were planned initially. The protein-coding genes were to be identified by synthesizing DNA complementary to messenger RNA, the complementary DNA or cDNA. The plan was to create a library of cDNA clones corresponding to all protein-coding genes in the human genome. At the time, the number of genes in the human genome was unknown, the estimates varying between 35,000 and 100,000 genes (Watson and Jordan 1989; Lander 2011). Finally, the goal of mapping the full DNA sequence of the human genome—that is, the sequence of its 3.2 billion bases—by 2005 was clearly highly ambitious, especially given that the critical breakthroughs in parallel sequencing technology came only after the completion of the HGP (Lander 2011).
A quick look at Table 2.1 shows the impressive achievements of the HGP over its first eight years and its goals for the final five years (Collins et al. 1998). The HGP finished in 2003, two years ahead of schedule. Through the HGP, we learned that the total number of protein-coding genes is much lower than expected (~21,000). The genetic map contained ~3,000 markers in 1994 but, in their 1998 report, Collins and colleagues planned to cover the genome with 100,000 single nucleotide polymorphisms (SNPs) over the next five years.4 In the same report, Collins et al. (1998) predicted that “SNPs will be a boon for mapping complex traits such as cancer, diabetes, and mental illness”, “… make possible genome-wide association studies…” and “… permit prediction of individual differences in drug response”.
Area | Goal 1993–1998 | Status as of October 1998 | Goals 1998–2003 |
---|---|---|---|
Genetic map | Average 2–5 cm resolution | 1 cm map published September 1994 | Completed |
Physical map | Map 30,000 STSs | 52,000 STSs mapped | Completed |
DNA sequence | Complete 80 Mb for all organisms by 1998 | 180 Mb human plus 111 Mb non-human | • Finish 1/3 of human sequence by end of 2001 |
• Working draft of remainder by end of 2001 | |||
• Complete human sequence by end of 2003 | |||
Sequencing technology | Evolutionary improvements and innovative technologies | 90 Mb/year capacity at ~$0.50 per base | Integrate and automate to achieve 500 Mb/year at ~$0.25 per base support innovation |
Capillary array electrophoresis validated microfabrication feasible | |||
Human sequence variation | Not a goal | _ | 100,000 mapped SNPs Develop technology |
Gene identification | Develop technology | 30,000 ESTs mapped | Full-length cDNAs |
Functional analysis | Not a goal | _ | Develop genomic-scale technologies |
E. coli complete sequence | Published September 1997 | – | |
Yeast complete sequence | Released April 1996 | – | |
C. elegans most of sequence | 80 % complete | Complete December 1998 | |
Drosophilia begin sequencing | 9 % done | Sequence by 2002 | |
Model organisms | Mouse: map 10,000 STSs | 12,000 STSs mapped | • Develop extensive genomic resources |
• Lay basis for finishing sequence by 2005 | |||
• Produce working draft before 2005 |
Since the completion of the HGP, a number of other genome-mapping efforts continue. For example, the International HapMap Project builds maps based on the combination of adjacent SNPs inherited together (haplotypes) and identifies SNPs that “tag” unique haplotypes; it is estimated that the human genome contains about 300,000–600,000 such haplotype-tagging SNPs, when compared with a total of 10 million common SNPs (hapmap.ncbi.nlm.nih.gov/thehapmap.html.en). Other efforts, enabled by the shift of parallel sequencing from the electrophoresis based to optical imaging based, focus on genome-wide epigenomic mapping of variations in chromatic modifications and methylation (Lander 2011).
In summary, genetics started in Mendel’s garden with the application of statistics, continued through the basic discoveries explaining the molecular and cellular mechanisms of the genetic machinery, and culminated in the mapping of human genome sequences and the cataloguing of inter-individual variations in its various features. The impressive achievements of the HGP, vis-à-vis basic knowledge about the human genome, advancements of genotyping technology and the creation of genetic databases: all set the stage for mapping the sources of inter-individual variability of complex traits, including the structural and functional properties of the human brain.
2.3 Cognitive Neuroscience
In 1861, Broca suggested in his report to the (French) Anatomical Society that the third frontal convolution of the left frontal lobe is the “seat” of “articulated” (spoken) language (Broca 1861). He reached this conclusion by evaluating the symptoms, and dissecting the brain, of a patient called Tan, a 50-year-old man who died in his (surgical) care, due to gangrene of a leg. The patient had been admitted to the Bicêtre Hospital 21 years previously, a few months after losing the ability to speak—since then, the only words he could utter were “tan, tan” (Text Box 2.3.). Broca performed an autopsy within 24 h of Tan’s death; he concluded that the centre of the pathology was in the posterior part of the third convolution of the left frontal lobe and suggested that this was the site of the original lesion that led Tan to lose his ability to speak, 21 years earlier (Broca 1861).
Text Box 2.3. The Patient Tan
Tan—a patient of Broca—was admitted to the Bicêtre Hospital a few months after losing the ability to speak. Reviewing medical records 21 years later (when he was treating Tan for gangrene), Broca put together a picture of a man who had been healthy and intelligent when originally admitted to the hospital, “who differed from a sane man only in the loss of articulated speech”, and whose brain pathology developed over the ensuing 21 years, affecting next the movement of his right arm then the right leg, eventually leaving him bedridden. But even on his death bed, “Tan understood almost everything that was said to him… Numerical responses were those that he could make the best, by opening or closing his fingers. I asked him many time how many days he had been sick? He [sic] responded sometimes five days, sometimes six days. For how many years had he been at Bicêtre? He [sic] opened his hand four times in sequence, and then pointed with a single finger; this would make 21 years, and one saw above that this information was perfectly exact” (Broca 1861).
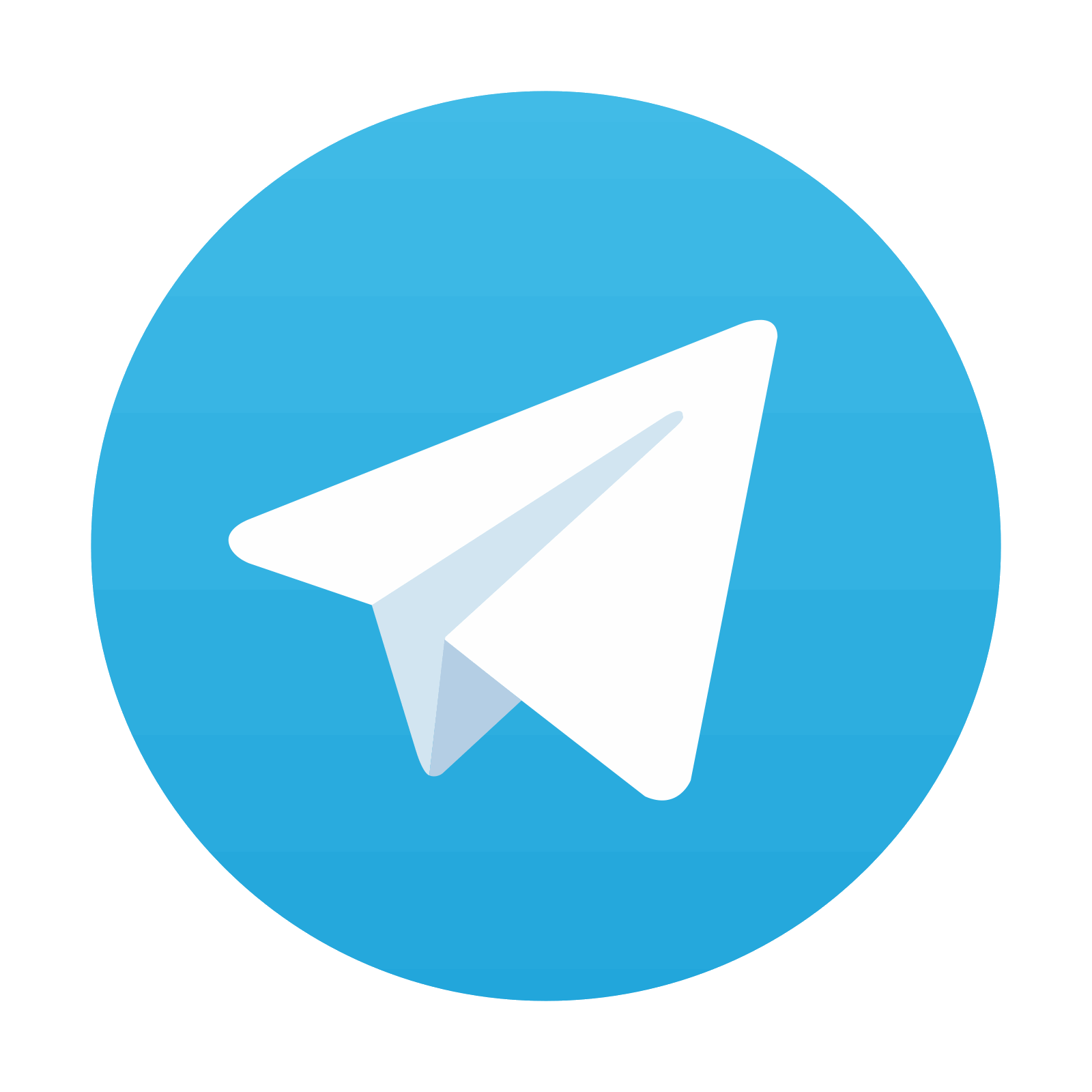
Stay updated, free articles. Join our Telegram channel
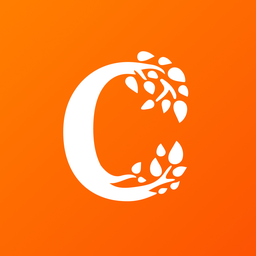
Full access? Get Clinical Tree
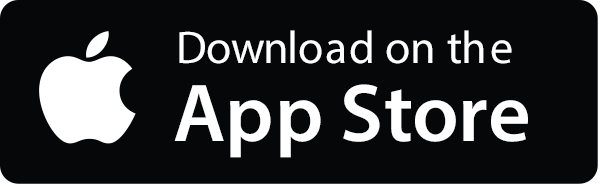
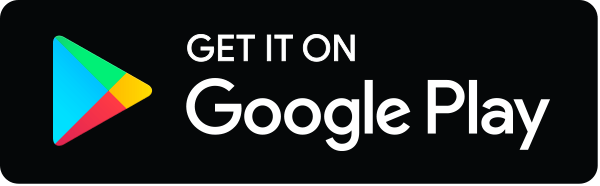