and Róbert Bódizs2
(1)
Institute of Experimental Medicine and Institute of Neuroscience, Budapest, Hungary
(2)
Semmelweis University Institute of Behavioral Science, Budapest, Hungary
Abstract
The significance of reactive (input-related) slow wave responses has remained hidden for a long time. The recognition of this phenomenon started with the discovery of K-complexes and continued with the description of the A1 phase of cyclic alternating pattern (CAP); however, their biological role and importance is not yet fully understood. We propose here that these phenomena represent an instant (short-term) slow wave homeostasis to ensure the necessary amount of slow waves of a night. We propose that this instant slow wave homeostatic regulation is a supplementary regulation tool of slow wave economy as a response to any perturbation threatening the realization of the necessary slow wave amount especially during the first cycles, when homeostatic sleep pressure is high. There we have the link between the arousal-related microstructural sleep regulation and the sleep slow wave homeostasis, believed hitherto to be different issues.
Keywords
CAPInstant slow wave homeostasisDelta injectionSensory evoked responsesK-complexBoosting of slow waves
While during wakefulness corticothalamic circuits react to a direct perturbation with a complex pattern of activation, during NREM sleep the only possible response is a stereotypical slow wave, that can be local or global, depending on stimulation intensity (Massimini).
6.1 CAP System and Sleep Homeostasis: Is CAP an Instant Homeostatic Tool?
The discovery of CAP in the mid-eighties by the Parma sleep research group (Terzano et al. 1985) and later studies have shown that this system is a very sensitive marker of NREM instability (Parrino et al. 2012). The distinction among different types of A phases (Terzano et al. 2000) and the contrasting functional behavior of A1 and A3 types set the stage for further development. The A1 type has been identified as sleep-promoting (antiarousal) event fueling slow wave sleep and behaving similarly as spontaneous slow waves. On the contrary, A3 has been identified as a PAT analogue, arousal-like event. These contrasting features of CAP constituents were well congruent with our earlier distinction of “synchronizational” and “desynchronizational” type microarousals. The different types of their distribution across the sleep cycles also support their different natures (Halász et al. 2004).
Terzano et al. (2005) analyzed how CAP is involved in the homeostatic and ultradian sleep dynamics. CAP A1 rate in NREM sleep undergoes a characteristic significant exponential reduction during night sleep parallel with the homeostatic decay of slow wave activity according to the Borbély’s S process (1982). This is congruent with our results on K-complexes (Halász 2005) showing similar time course across the sleep process. The same effect of the homeostatic process has been shown by Vyazovskiy et al. (2009) finding the alteration of the amplitude of slow waves, elicited by intracortical electrical stimulation during NREM sleep (see Chap. 7). Ferri et al. (2005) have shown that CAP A1 is involved in the gradual synchronization of EEG during sleep. In contrast to this, A2–3 rates have shown quite different distribution with cyclic peaks before the REM periods on the ascending (“A”) slopes of cycles, without any dampening during the course of sleep (Fig. 6.1). This means that input-dependent A1 events have a part in building up slow wave sleep and follow the course of the homeostatic process. A2–3 events, on the contrary, belong to the wake/REM-promoting system taking part in arousal regulation of NREM sleep.
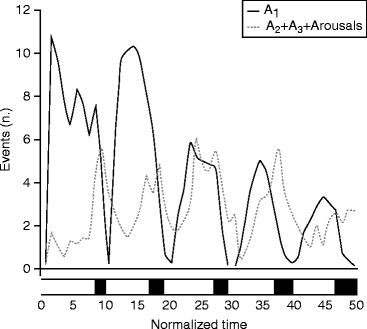
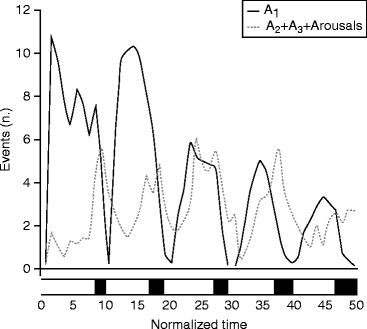
Fig. 6.1
Involvement of CAP A subtypes in the homeostatic and ultradian regulation of sleep. CAP A1 events follow the dampened sinusoid oscillation reflecting the exponential decrease in slow waves as predicted by the two process models of sleep regulation of Borbély. On the other hand, CAP A2–3 subtypes and arousals are peaking shortly before REM sleep (black boxes at the bottom of the figure) without showing any sleep-dependent decrease (Terzano et al. 2005)
As mentioned earlier, the CAP system was considered by the Parma group as a marker of sleep instability on one hand and as a buffer system against perturbations of NREM sleep on the other. Both seem true, but the “instability” aspect is more attached to the dynamics of A2–A3 events, and the “buffer system” aspect is more attached to the dynamics of the A1 events. Similarly to slopes of sleep cycles exhibiting double nature “sleep promoting” during the descending and “wake/REM promoting” during the ascending slopes, CAP constituents follow the same alternating trend (Fig. 6.1).
Furthermore, if we try to check the biological role of the CAP system, the “instability marker” role seems to be a medical consideration, emphasizing abnormality as something deviating from the physiological. We propose to look on the other side of the coin and rather examine the “buffer system” aspect. If we do so, a very interesting feature of the CAP system is coming to light. We may consider the system as a short-range homeostatic process in which the amount of slow wave activity is instantly buffered and sleep continuity is kept preserved. We may comprehend the CAP system as a natural “delta injection,” fuelling the defense of sleep against perturbations.
With the recognition of the CAP A1 features, we have learned that the flow of slow waves is not continuous even along the descending slope of the first cycles, but appears in input-related bouts. Depending on the degree of sleep perturbation, 30–40 % of the sleep time is spent usually in CAP A phase (Terzano et al. 2000). The CAP sequences are characterized by recurrent bouts of reactive slow activity (main frequency 0.25–2.5 Hz) represented by the A1 phase amounting more than 60 % of all the CAP sequences. This rate is an overall sleep average. The participation of slow waves increases in deep sleep and the range of A1 type phases among the A subtypes also increases; thus, the specific rate of reactive slow wave activity in deep sleep is much higher.
A not much emphasized aspect demonstrated by the CAP phenomenon is the input-related nature of an important part of NREM sleep slow wave activity. If a sensory stimulus is applied during B phase, an A phase event is elicitable. The type of this A phase response depends on the actual sleep pattern receiving the disturbing stimulus according to cycle, stage, and slope orientation.
So we have to realize that an important compartment of the slow wave cloud over the sleeping brain has an input-related origin linked to the CAP system. The Terzano study, connecting the A1 type with the homeostatic process, enlightens another important aspect, namely, A1 power reflects the actual level of the homeostatic decay of slow waves; consequently, it is cortical (metabolic) demand dependent.
The early experiments of the Parma group (Terzano et al. 1988) clearly showed that the slow wave power of A1 and A2 phases increased proportionally under the increasing sound pressure levels of 45–75 dB white noise; the fast frequencies’ power during phase A3 remained unchanged. So the increase of the reactive slow wave rate seems to be proportional with the degree of sleep perturbation caused by external sound (Fig. 6.2).
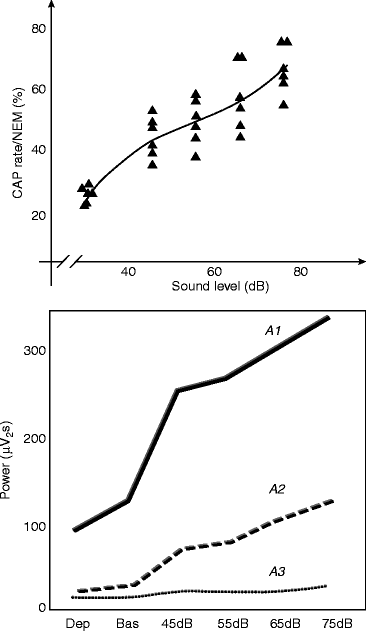
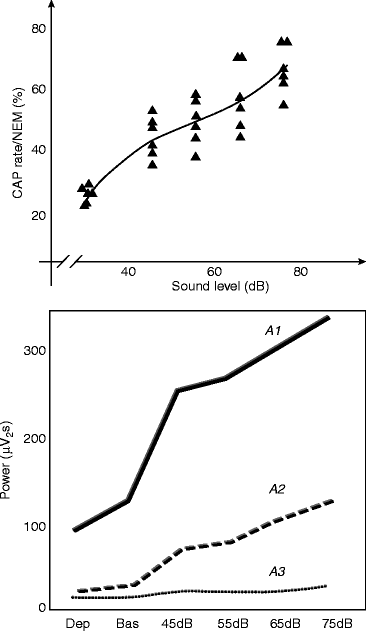
Fig. 6.2
The input and sleep pressure dependence of the cyclic alternating pattern (CAP). Top: Increasing level of sound (acoustic stimulation with white noise) lead to increases in CAP rate. Bottom: Power of the CAP A1 and A2 subtypes (containing slow waves) increases with increasing levels of sound (acoustic stimulation with white noise), while power of A3 (containing fast EEG activity mainly) remains relatively unchanged. Sleep deprivation decreases the power of input-related (reactive) delta bouts. Dep deprivation, Bas baseline
When sleep delta power increases after a sleep-deprived night, the CAP system reacts with a robust decrease of its rate (De Gennaro et al. 2002). The supplementation of sleep with slow waves during the night after sleep deprivation obstacles the production of CAP sequences: probably because the sleep-promoting system is being saturated.
We have obtained similar results on K-complex production under the influence of random sound stimulation (Halász 1982, page 70). During the stimulation nights, the frequency of K-complexes (spontaneous + evoked) has increased, while the number of spontaneous ones decreased (Fig. 4.3). Interestingly, when the frequency of sound stimuli was further increased (until 17/min), the elicitability of K-complexes broke down, while the slow wave power of the background activity increased (Fig. 6.3).
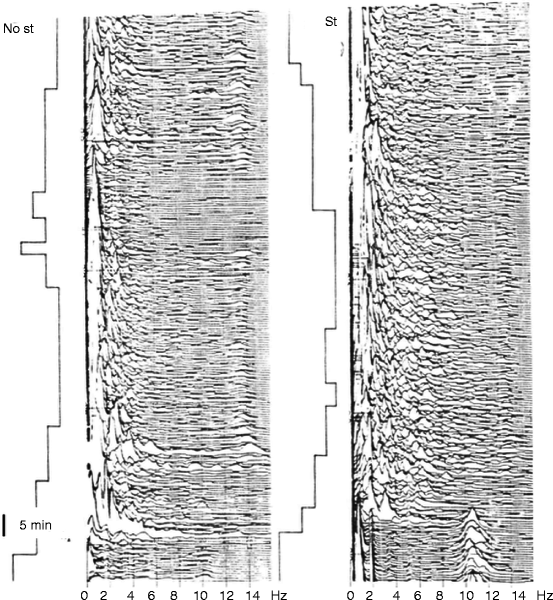
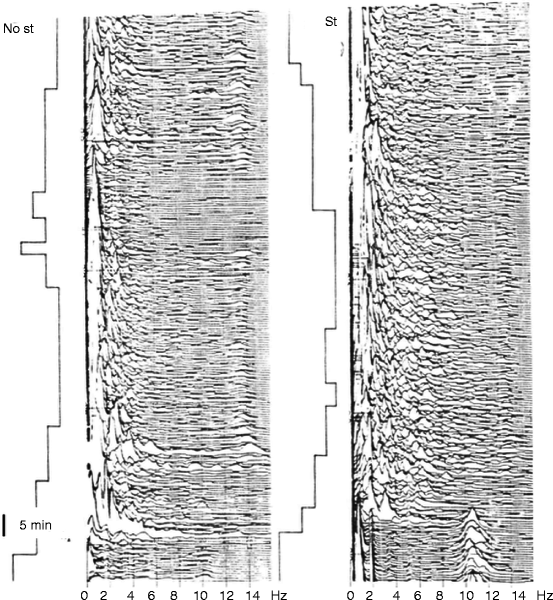
Fig. 6.3
Compressed spectral array of one cycle of NREM sleep EEG. Left: spontaneous sleep. Right: acoustic stimulation at the frequency of 17/min. Under stimulation power of slow wave activity increased. St stimulation, No St no stimulation
Here, again we are emphasizing that the input-dependent slow wave supplement within the frame of the CAP system provides an instant homeostatic function that ensures the necessary sleep protection by allowing lability (and reversibility) instead of bistability, as proposed by Saper et al. (2001).
An important feature of the instant homeostatic role of CAP A1 phases is their timing, which is mainly limited to the descending slopes of the first cycles during homeostatic decay of slow wave production. This time window seems to be the main possibility to get the necessary amount of slow waves: if arousing events decrease slow wave production, the CAP system provides supplementary slow waves. The supplementation of sleep with slow waves during the night after sleep deprivation obstacles the production of CAP sequences: probably because the sleep-promoting system is being saturated. Another also important feature of the instant homeostatic function is that it is targeted to the frontal lobe as was shown by Ferri (2006). Therefore, it seems that the frontal lobe is under a double homeostatic regulation, probably because this sensitive, phylogenetically new region needs slow wave activity for the recuperation of cognitive functions processed mostly here.
Presently, we do not know whether this is due to the characteristics of input factors (left dominance, e.g., might be associated with the greater metabolic demand in the cortical areas of the speech network) or to the sensitivity (amount of restitutional need) of certain cortical areas (e.g., frontal areas responsible for more sensitive human cognitive neofunctions).
We should remind the reader about the earlier touched distinction between tonic and phasic regulation. So we may assume that here, similarly to other physiological regulations, again a double, tonic, and phasic regulation works together. The traditional long-term (exhibited during the next sleep period) homeostatic regulation is a slow chemically driven process, contrasting to the short-term (instant) slow wave regulating homeostatic process which is faster and more synaptically driven. Beyond the differences, the two homeostatic processes are interrelated: the instant process is built upon the traditional one that borrows force from the strength of the homeostati- cally tuned thalamocortical system and is timed to the high homeostatic pressure period of the cycle. The common subject of the double homeostasis, the slow wave activity.
As it was enumerated in the previous chapters, more and more evidences support the view that NREM sleep, and especially slow wave sleep homeostatic regulation, is in the same time governed by use-dependent plasticity processes. In other words, slow wave homeostasis and use-dependent plasticity seem to be closely related, supporting the restitution of those cortical areas which have the highest vulnerability, engaged with cognitive functions.
Based on results of contemporary sleep research, the regulation of NREM sleep can be integrated in a more uniform concept as earlier. Arousal regulation, homeostatic regulation, and plastic changes of sleep all can be viewed as complex relationships around reactive use-dependent regional slow wave activity of sleep.
6.2 Mechanism of Sleep-Like Response in NREM: Relationship with the Late Slow Wave Components of Sensory Evoked Responses
The precise mechanism of input-related (reactive) slow wave responses either in the form of K-complex or CAP A1 phase is presently unknown, but we may delineate some of the constituents of the complex mechanism underpinning this response.
To understand more, first we should discuss the transfer of information from sensory input to cortical perception during sleep compared to the waking state. Sensory systems reacting to environmental stimuli convey impulses to the thalamus and from the thalamus to the cortex. During the waking state, the complete message of the peripheral receptors reaches the sensory cortical areas: the input and output ratio of the thalamic cells is almost one (Coenen 1995). During sleep from drowsiness to deep NREM sleep, due to the decreasing output rate of the more and more hyperpolarized thalamic relay cells as spindling and later slow wave sleep develops, the transfer ratio falls down progressively to 0.3–0.4. Several human studies showed that although sensory inputs after peripheral stimulation pass the thalamic gate during all phases of NREM sleep, due to the burst-firing working mode of the thalamocortical system, the membrane potentials fluctuate both in thalamic and cortical neurons, and during the down state of cortical slow oscillation, the sensory processing becomes nonstationary and impaired (Massimini and Rosanova 2003; Rosanova and Timofeev 2005; Schabus et al. 2012).
Studies on evoked responses during the wake-sleep cycle have a long-lasting history since 1939 (Davis et al. 1939). The sensory evoked potentials (ERP) are divided to early and late components. The early waves are determined by the physical qualities (intensity, duration) of the stimulus, while the late ones are influenced by endogenous factors related to the elaboration of the sensory information. Late components are particularly sensitive to vigilance changes. The increased synchronization in slow wave sleep due to the gating by burst-firing thalamocortical neurons contributes to the enlargement of the late ERS components, taking shape of large polyphasic slow waves. The most frequently studied evoked potential in sleep is the auditory evoked potential.
In a previous chapter, we enumerated arguments for K-complex being considered analogous with the late nonspecific components of evoked potentials in NREM sleep. The recent fMRI work of Riedner et al. (2011) has confirmed this relationship.
The functional relationship of K-complex components with slow wave oscillation of NREM sleep was evidenced congruently from different aspects (Plihal et al. 1996; Amzica and Steriade 1998; Peszka and Harsh 2002; Nicholas et al. 2002; Halász 2005; Cash et al. 2009). Nowadays, it is clear that sensory stimuli “act as a trigger pulse producing a resonance in the synchronized neural assembly” of slow sleep oscillation (Coenen 1995). In a recent work, the entrainment of the slow oscillation of auditory thalamic neurons was explicitly proven by repetitive sound stimuli (Gao et al. 2009) which were able to drive the timing of cortical up-down state oscillation during slow wave sleep.
By recording intracortical field potentials by chronically implanted multielectrodes in freely moving cats, Karmos et al. (1988) have found that the middle latency positive-negative wave that followed the early components of acoustic evoked responses was highly dependent on the level of vigilance. Its amplitude and duration gradually increased during the progress of slow wave sleep. Parallel registered unit activity showed a marked reduction during the deep-positive slow wave, between 25 and 100 ms. The length of this inhibitory period was in close correlation with the depth of slow wave sleep. Working later with an advanced variation of their cortical multi-microelectrode, Grand (2010) repeated in his Ph.D. work these experiments and found essentially the same: cessation of unit activity during the slow wave specific deep-positive/surface-negative slow component, which he interpreted as “evoked down state” (Fig. 6.4). According to his opinion, “information processing in the cortex during sleep is inhibited by the evoked down states that block the information processing in the 25–100 ms time interval after the stimuli and prevent from awakening if the stimulus is irrelevant. On the global cortical scale, human K-complexes act similarly as the evoked down states in the cat auditory system, implicating that sleep protecting mechanisms may essentially be uniform across species.”
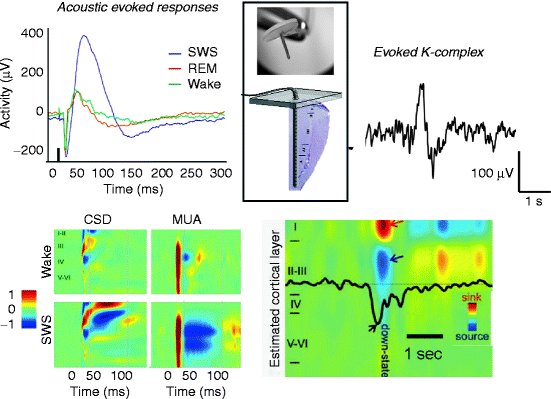
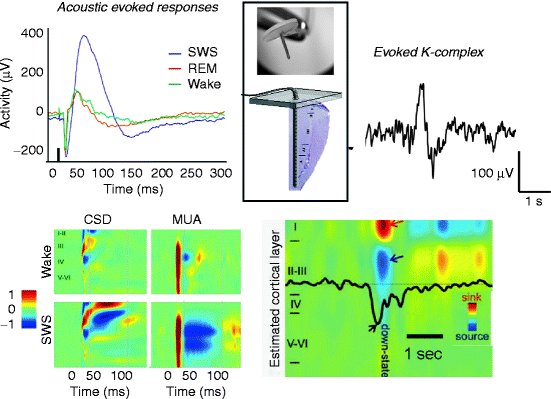
Fig. 6.4
Evoked down states in acoustic evoked responses (cats) and K-complexes (humans). Acoustic evoked responses of cats (Grand 2010) and K-complexes of humans (Cash et al. 2009) were registered by subdural grid and multi-microelectrode (thumbtack) with 24 contacts at 150 μm intervals (upper part of the insert). The track of the electrode across the cortex is shown in the lower part of the insert. Colored maps show intracortical distribution of current and unit activity according to cortical layers. Red indicates inward currents (sink) in CSD and increase in neuronal firing in MUA. Blue shows outward currents (source) in CSD and decrease in neuronal firing in MUA. Black arrow indicates decreased neuronal firing in the case of evoked K-complex. SWS slow wave sleep, CSD current source density, MUA multiunit activity
Congruent with the results of Riedner et al. (2011), this work supports the possibility that sensory evoked potentials, and among them especially those evoked by acoustic stimuli, together with a surface-negative slow wave component, exhibits a stop of cortical cellular activity during NREM sleep. This period of interrupted firing lasts in the acoustic cortex from 25 to 100 ms after the stimulus. This component probably reflects an entrainment of the thalamic inhibitory network and by the thalamocortical and cortico-cortical recruitment probably contributes to the development of the 350–550 negative slow component of K-complex on the level of the macro-EEG.
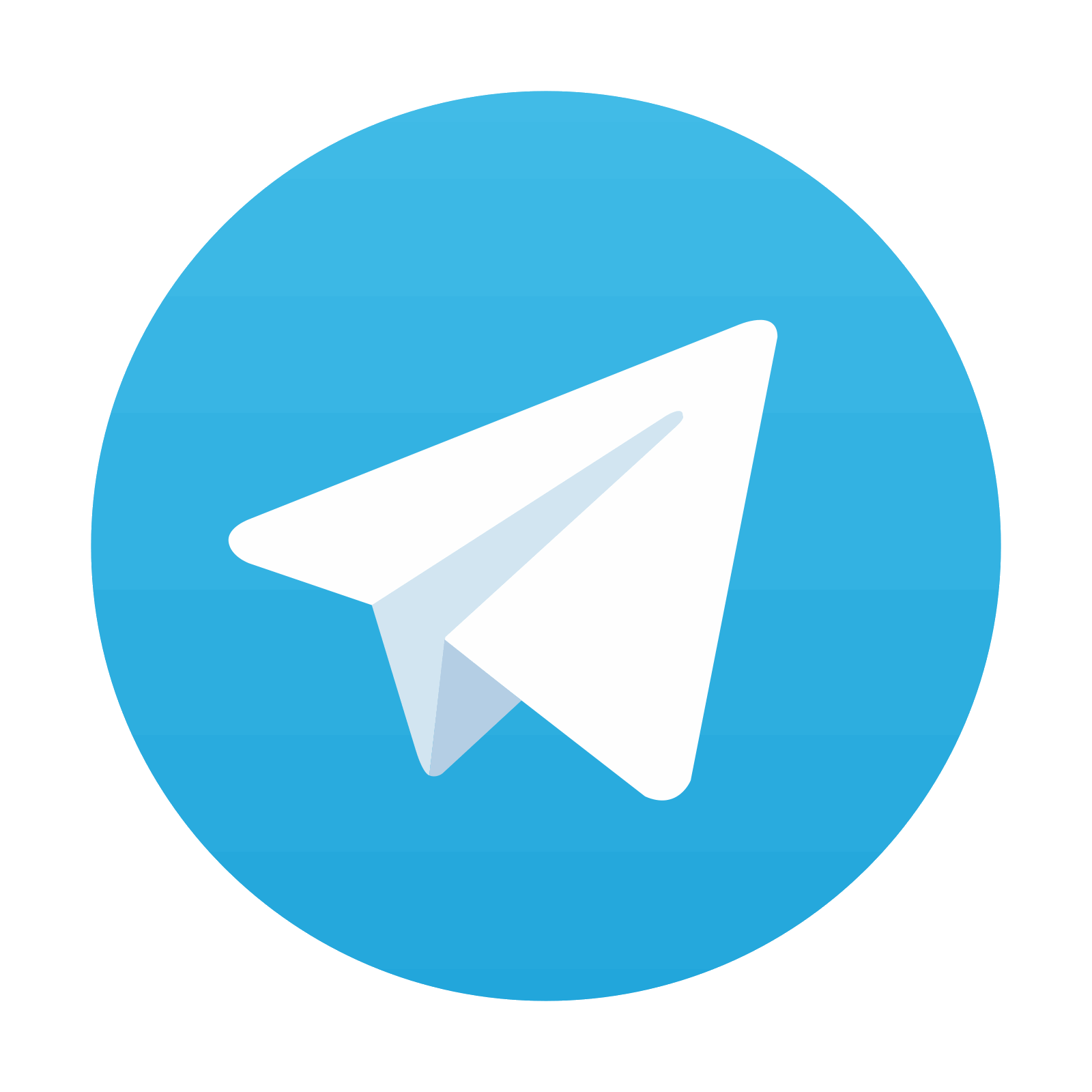
Stay updated, free articles. Join our Telegram channel
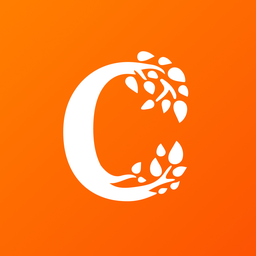
Full access? Get Clinical Tree
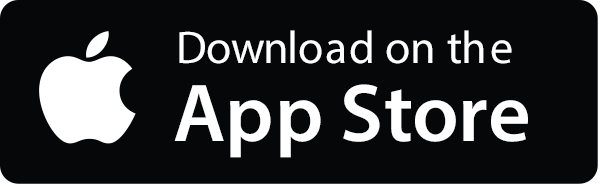
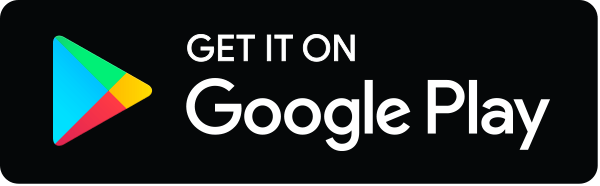