Fig. 5.1
Hominin brain size evolution. Endocranial volume (EV) in milliliters is plotted against geological age of hominin fossils (mya, million years ago): gracile Australopithecines (green triangles), robust Australopithecines (black triangles), Homo sp. (light blue circles; includes the earliest representatives until Neanderthals and H. floresiensis), H. sapiens (dark blue squares). MH 1 is A. sediba, LB 1 is H. floresiensis. Data from Holloway et al. (2004), Falk et al. (2005), Carlson et al. (2011)
However, body size changed in the same time period and brain size is expected to be not independent of overall body size. Relating brain size to body size, Ruff et al. (1997) argued that a period of stasis until about 6,00,000 years ago preceded the major increase of relative brain size during the Middle Pleistocene that continued until about 1,50,000 years ago. Neanderthals, although having larger brains in absolute terms but also larger bodies, were slightly less encephalized than early anatomically modern humans as well as humans living today (Ruff et al. 1997).
The discovery of new hominin fossils on the island of Flores, Indonesia, dating to quite recent times and described as a novel species named H. floresiensis (Brown et al. 2004; Morwood et al. 2004), brought forward unexpected evidence for hominin brain size evolution. The most complete individual of this population, LB 1, has lived about 18,000 years ago during a time at which paleoanthropologists thought H. sapiens was the only remaining hominin species (Morwood et al. 2004). LB 1 shows a combination of primitive and derived features including an endocranial volume of only 417 ml (Falk et al. 2005). The small brain size, besides other features, led to the alternative hypothesis that these fossils do not represent a new species but are modern human individuals who suffered from various pathologies, for example microcephaly (e.g., Jacob et al. 2006; Martin et al. 2006; Vannucci et al. 2011). It is a long way to go until the controversies about these two opposing hypotheses are settled but Falk et al. (2005, 2007, 2009) presented several analyses based on different samples to argue that, although the size of the brain is incredibly small, its shape resembles that of H. erectus and groups LB 1 not with human individuals suffering from microcephaly.
The overall trend of increasing brain size during hominin evolution coincides with an increase of technological sophistication and behavioral changes as apparent from the archeological record (Klein 2009). Therefore, brain size has been related to cognitive and behavioral abilities. This makes sense because brain size to some extent reflects neural density, and the amount of neural wiring and connectivity that underlie brain function. Among primates, humans have the largest brain and the most complex behaviors and human individuals affected by diseases that involve small brain sizes (like microcephaly) often suffer from cognitive impairments. However, H. floresiensis, for example, is associated with stone tool artifacts although they had very small brains (Morwood et al. 2004; Brumm et al. 2006; Moore et al. 2009). Furthermore, among healthy humans living today, brain size is only very loosely correlated to any measure of intelligence (e.g., Schoenemann et al. 2000). To summarize, brain size is a rough proxy for cognitive and behavioral capabilities but within-group correlation is low.
It is worth noting here that, among mammals, brain size is correlated to body size in a non-linear (allometric) way (e.g., Gould 1975). The encephalization quotient (Jerison 1973) and the index of progression (Bauchot and Stephan 1966), for example, are ratios between observed brain size and expected brain size for a given body size, that are computed to better interpret encephalization in terms of cognition and intelligence. However, these kinds of indices might be very different depending on the taxonomic level that is used for their computation. Alba (2010) discusses these issues in detail.
Developmental Increase of Endocranial Volume in Humans and Chimpanzees
To evolve a large brain, it has to grow more during an individual’s ontogeny, either through increased growth rates or/and through prolongation of brain growth. For the interpretation of such ontogenetic patterns, brain size has to be related to age. A growth curve is an empirical model of brain size increases with age and can be formalized using regression analysis.
Ideally, empirical data for brain size would be measured in a growing individual in frequent intervals resulting in brain size measurements of one individual with associated age information. These longitudinal data are unfortunately rare. However, head circumference is measured repeatedly during regular medical examinations for babies and is used as approximation for brain size to evaluate brain growth. Only recently, larger samples of longitudinal MRI (magnetic resonance imaging) scans of brains have become available and will be of help for future research (e.g., Knickmeyer et al. 2008). More frequently, endocranial volume is measured in a sample of differently aged individuals. The individuals of such cross-sectional samples also show individual variation in brain size that is not directly related to age. However, it can be assumed that the average growth curve based on a large cross-sectional sample is a good representation of a group’s general brain growth pattern (e.g., Leigh 2004).
For species living today, other variables are available in addition to endocranial volume: brain weight and brain volume itself. Brain weight can be measured during autopsies and brain volume is measured from MRI scans, either after death or in vivo. It is worth noting that endocranial volume and brain weight or brain volume are not directly comparable. Cerebrospinal fluid, meninges, blood vessels and cranial nerves take up room in the cranial cavity, and there are some age-related changes of the proportion of cerebrospinal fluid (Wanifuchi et al. 2002; Rengachary and Ellenbogen 2005; Hublin and Coqueugniot 2006; Coqueugniot and Hublin 2012). The amount of cerebrospinal fluid measured together with the brain is sometimes difficult to control for during autopsies and the relationship between brain volume and brain weight can be biased by pathologies (Ho et al. 1981; Coqueugniot and Hublin 2012). There are advantages and disadvantages regardless of whether brain weight, brain volume or endocranial volume is used, but most importantly, these variables should not be mixed in a single analysis.
Figure 5.2 illustrates brain growth patterns. At birth, human neonates have a brain weight of about 374 g (mean of 48 newborns, Bischoff 1880; Marchand 1902) while the brain of chimpanzee newborns weighs only 151 g (mean of 22 newborns; Herndon et al. 1999; DeSilva and Lesnik 2006). Hüppi et al. (1998) measured brain volumes from in vivo MRI to be about 400 ml in human neonates. The mean endocranial volume as measured from 15 virtual endocasts is about 310 ml for human neonates (Coqueugniot and Hublin 2012) and 127 ml for one chimpanzee neonate (Neubauer et al. 2012). Since human gestation is slightly longer than in chimpanzees (38 weeks in humans versus 34 weeks in chimpanzees; Kappeler and Pereira 2003), the higher neonatal brain size in humans is partly rooted in the duration of prenatal brain growth but mostly because of higher fetal growth rates, or in other words faster brain growth before birth. This difference is not surprising given the differences in body size and the differences in adult brain weight and endocranial volume (1335 g or 1330 ml in humans versus 382 g or 379 ml in chimpanzees; Bischoff 1880; Holloway et al. 2004; DeSilva and Lesnik 2006; Neubauer et al. 2012). Therefore, neonatal brain size is often expressed as a percentage from the adult mean value (proportional brain size or proportional endocranial volume).
Most studies agree that humans achieve a smaller percentage of their total brain growth prenatally than chimpanzees and other primates (Schultz 1940; Schultz 1941; Count 1947; Jordaan 1976; Gould 1977; Passingham 1982; Martin 1983; Dienske 1986; Smith and Tompkins 1995; Leigh 2004; Coqueugniot et al. 2004; DeSilva and Lesnik 2006; Hublin and Coqueugniot 2006; DeSilva and Lesnik 2008; Coqueugniot and Hublin 2012) but a few other studies (Fragaszy and Bard 1997; Fragaszy et al. 2004; Kennedy 2005; Vinicius 2005) argue for comparable or even identical proportional brain sizes at birth. DeSilva and Lesnik (2006) used a resampling technique to address potential problems due to the nature of cross-sectional samples and attempted thereby to solve the contradictory results. They related every neonate of their sample to every adult individual and interpreted the range of percentages they obtained. It is worth noting that this resampling approach artificially increases the variation around the mean growth curves, resulting in an artificially increased overlap between humans and chimpanzees. This is because the resampling includes pairings of the smallest neonatal brains to the largest adult brains and vice versa, although brain size at birth is related to adult brain size as can be seen from head circumference data (used as proxy for brain size) of 15-year-olds that is strongly correlated to their head circumference at one year of age and even earlier (Coqueugniot et al. 2005; Hublin and Coqueugniot 2006). Despite this fact, DeSilva and Lesnik’s (2006) resampling approach strongly supports the claim that there is a real difference of proportional brain size at birth. While they found that chimpanzees have achieved 40 ± 6 % of adult brain weight, the human brain at birth has reached only about 30 ± 5 % of its adult size (DeSilva and Lesnik 2006). Furthermore, it should be noted that humans have a smaller proportional brain size at birth than chimpanzees regardless of whether brain weight data or endocranial volume data are used but that the exact numbers are slightly different. Coqueugniot and Hublin (2012), for example, reported an average endocranial volume of 310 ml at birth in humans (n = 15), which is about 22 % in relation to adult endocranial volume, and Neubauer et al. (2012) found a proportional endocranial volume of 34 % for a newborn chimpanzee that died a few days after birth and of 58 % for a 66-days-old chimpanzee.
Brain size data of differently aged individuals after birth allow insights into postnatal brain growth. The overall pattern of the brain growth curve is similar in humans and chimpanzees (see Fig. 5.2): perinatally, growth rates are high for some time and then decrease until the amount of the growth rate cannot be distinguished anymore from the variation between individuals of the cross-sectional samples. Leigh (2004) reports growth rates of about 600 g/year for human newborns. This rate is decreasing quickly until about 2 years of age. At this age, growth rates decrease slower until adult brain size is achieved at about 5 to 6 years. In chimpanzees, growth rates at birth are about 100 g/year and also decrease until about 2 years. After this age, the chimpanzee brain grows under about 25 g/year until at about 4–5 years adult size is achieved (Leigh 2004). When growth rates are related to brain weight at a given age, the pattern is more similar, mostly only differing in the first year of life in which humans have far higher relative growth rates than chimpanzees (Leigh 2004). Some authors (Holt et al. 1975; Gould 1977; Martin 1983; Smith and Tompkins 1995; Coqueugniot et al. 2004; Hublin and Coqueugniot 2006) suggested a significantly prolonged postnatal brain growth in humans as compared to chimpanzees while others (Jolicoeur et al. 1988; Vrba 1998; Rice 2002; Leigh 2004) suggested modest differences that can be neglected. Leigh (2004) argued that higher growth rates are the primary cause for the postnatal surplus of brain size increase in humans and that a prolongation of brain growth plays only a secondary role. However, both might be important, differences in growth rates and differences in the duration of growth (Coqueugniot et al. 2004; Hublin and Coqueugniot 2006; Neubauer and Hublin 2012; Coqueugniot and Hublin 2012; Neubauer et al. 2012). Improved samples of ontogenetic data will clarify the contradictory interpretations in the future.
The small proportional brain size at birth in humans has been linked to another feature of modern humans. The obstetric dilemma hypothesis states that our efficient bipedal locomotion requires a narrow pelvis (Lovejoy et al. 1973), while a large brain and therefore a large skull at birth requires a broad birth canal and consequently a broad maternal pelvis. In contrast to chimpanzees and the other great apes that have spacious birth canals, the head of a human newborn fits tightly through the birth canal (Trevathan 1987; Rosenberg and Trevathan 1996; Rosenberg and Trevathan 2002). Therefore, it was suggested that the human brain grows prenatally to a maximum size so that it just fits through the birth canal and consequently that brain growth is shifted to the time after birth. The anatomical modifications of efficient bipedal locomotion and the aim to grow the brain as much as possible prenatally, probably due to energetic advantages, even required the evolution of a dangerous rotational birth mechanism (Rosenberg and Trevathan 2002).
The shift of brain growth from pre- to postnatal periods, no matter if related to the evolution of bipedalism, has implications for energy allocation and life history strategies, although it is difficult to categorize humans within the strategies known for primates (Leigh 2004). However, as a species of “cooperative breeders,” a mother can distribute the costs of nursing an infant across several members of the group to meet the metabolic costs of an infant’s postnatal brain growth. This allows for an early weaning age and therefore a shorter inter-birth interval (Hrdy 2007; Sear and Mace 2008) although the energetic requirements for our prolonged brain growth are high for a long time after birth. The discussion about the relationship of brain growth with life history patterns and energy allocation merits a review of its own and goes beyond the scope of this paper. However, given the high energetic costs, there have to be serious advantages to grow (and to evolve) a large brain (see for example Mink et al. 1981; Holliday 1986; Leonard and Robertson 1992, 1994, 1997; Dewey et al. 1993; Aiello and Wheeler 1995; Martin 1996).
It has been further hypothesized that the human brain growth pattern (duration, timing, and growth rates) is advantageous for cognitive development (e.g., Ragir 1985; Huttenlocher 1990; Smith and Tompkins 1995; Rosenzweig and Bennett 1996; Coqueugniot et al. 2004). This specific pattern brings forth an extended period of high neuronal plasticity, or in other words a large portion of brain development occurs while we already interact with the extra-uterine environment. The underdeveloped brain seems to be more susceptible to the influence of postnatal experiences including social interactions and other external stimuli. The morphological development of the brain and cognitive development are tightly and complexly interrelated. Support for this hypothesis comes from studies of brain development related to cognitive development (e.g., Sowell et al. 2003; Gogtay et al. 2004; Sowell et al. 2004b; Sowell et al. 2004a; Nagy et al. 2004; Durston et al. 2004; Brown et al. 2005; Casey et al. 2005; Shaw et al. 2006) and clinical studies of children who suffer from dysfunctions of brain development that are related to cognitive impairments (e.g., Courchesne et al. 2001; Carper et al. 2002; Courchesne et al. 2003; Dementieva et al. 2005; Hazlett et al. 2005; Dawson et al. 2007; Mraz et al. 2007; Courchesne et al. 2007; Webb et al. 2007; Schumann et al. 2010). While cognitive development cannot be analyzed in extinct species, the fossil record provides evidence on endocranial growth patterns.
Evolutionary Changes of Brain Growth
The available sample of Neanderthal remains is the largest of any extinct hominin group. It includes several variously aged individuals so that ontogenetic patterns can be investigated in this cross-sectional sample. Still, the sample is rather small so that estimating a brain growth curve bears some uncertainties.
Cranial remains of two neonates are preserved well enough to reconstruct their endocasts and estimate their endocranial volumes. The Mezmaiskaya baby is an about 1 to 2 week-old individual that was found in a cave in the northern Caucasus, Russia (Golovanova et al. 1999; Ponce de León et al. 2008). Its cranial remains yielded mitochondrial DNA that definitely identifies this individual as a Neanderthal (Ovchinnikov et al. 2000). The second Neanderthal baby is Le Moustier 2. This individual was found already about 100 years ago in the Dordogne in southwest France, fell into oblivion and was rediscovered only in 2002 (Maureille 2002). Ponce de Léon et al. (2008) reconstructed the cranium of the Mezmaiskaya baby and estimated that its endocranial volume was between 422 and 436 ml. Assuming an age of 1 to 2 weeks when this individual died and similar growth rates as in modern humans, Ponce de Léon et al. (2008) estimated a neonatal brain size of 382 to 416 ml. An alternative reconstruction of the Mezmaiskaya baby by Gunz et al. (2012) yields a similar, only slightly smaller endocranial volume estimate between 414 and 423 ml. Gunz et al. (2010) reconstructed the cranium of the second Neanderthal baby, Le Moustier 2, and estimated the endocranial volume of this individual to be between 408 and 428 ml. Ponce de Léon et al. (2008) and Gunz et al. (2010, 2012) therefore concluded that absolute brain size at birth in Neanderthals was similar to brain size of modern human babies. However, this is only true when brain volumes derived from MRI scans (Hüppi et al. 1998) but not when endocranial volumes measured from endocasts (Coqueugniot and Hublin 2012) are used as human reference value.
Based on an average Neanderthal adult brain size of 1,498 ml (Holloway et al. 2004), the proportional endocranial volume at birth ranges between 25 and 29 % (this range includes Mezmaiskaya’s endocranial volume as measured and as extrapolated from a two-week-old individual to a true newborn). Proportional endocranial volume at birth is therefore slightly higher than for modern humans (22 %, Coqueugniot and Hublin 2012) probably due to the larger average brain size for adult Neanderthals.
The postnatal growth curves of modern humans and Neanderthals are quite similar. Coqueugniot and Hublin (2007) found that proportional endocranial volume in nine Neanderthal juveniles is in the upper range of the modern human growth curve. Ponce de León et al. (2008) suggested that the higher absolute endocranial volume in Neanderthal adults resulted from slightly higher growth rates during early infancy, given that the duration of brain growth seems to be comparable between Neanderthals and modern humans. Alternatively, it can be hypothesized that the Neanderthal brain was already slightly larger at birth and continued to be in the upper range of modern human variation during the entire course of postnatal ontogeny until adulthood (see endocranial volume data for neonates in Coqueugniot and Hublin 2012). Resolving this question rests on improving high quality data for recent humans rather than improving Neanderthal data.
It is worth noting that we need not only to estimate the endocranial volume of fragmentary fossil individuals to evaluate brain growth patterns of extinct taxa, but also their age at death. This is usually done by interpretation of dental calcification and eruption patterns based on a model of an extant species. Dental microstructures like enamel and dentine that are formed with a consistent repeat interval during development are nowadays used to determine age of death more precisely (e.g., Smith 2004; Smith 2006). Using these techniques, Smith et al. (2010) among others suggested that Neanderthals consistently had a slightly faster dental maturation than modern humans. If so, this implies that Neanderthals had higher growth rates and shorter brain growth duration.
For H. erectus, the fossil record includes one relevant immature fossil to evaluate brain growth, the Mojokerto individual from Java dated to about 1.8 million years (Von Koenigswald 1936; Swisher et al. 1994). Coqueugniot et al. (2004) estimated that this individual has an endocranial volume of 663 ml. Unfortunately, dental remains are not preserved that could be used to estimate Mojokerto’s age but the developmental states of the area around the frontal fontanel and the subarcuate fossa suggest an age between 0.5 and 1.5 years (Coqueugniot et al. 2004). Using contemporary H. erectus adults to compute a proportional brain size, Mojokerto achieved around 87 % of adult brain size. Therefore, the growth pattern of this individual was more chimpanzee-like than human-like (Coqueugniot et al. 2004). However, Mojokerto’s absolute endocranial volume falls within the modern human variation (Leigh 2006). Based on his estimation of neonatal brain size in H. erectus, Leigh (2006) suggested that the human-like pattern of rapid early brain growth was already established in H. erectus. Anyhow, given the differences in adult brain sizes of several hundred milliliters between modern humans and H. erecuts, the brain growth pattern of the latter has to be different to the one in modern humans but the quality of data at this point does not allow determination of how they differ exactly.
Several immature individuals from earlier hominin species are known and can be used to determine their endocranial volume. These specimens include for example the Taung child, an Australopithecus africanus that lived about 2.5 million years ago in South Africa (Dart 1925), or the Dikika baby, an Australopithecus afarensis that lived about 3.3 million years ago in Ethiopia (Alemseged et al. 2006). However, it is obvious from the preceding discussion on H. erectus that it is difficult to interpret brain growth patterns based on a single immature individual, far more so for older fossils for which, for example, it is even harder to estimate age at death.
To summarize, there is a lot to learn from brain growth patterns in fossil hominins, but the available ontogenetic data are rare and prone to uncertainties. Our closest extinct relatives, the Neanderthals, had very similar growth patterns as compared to modern humans, although there could be relevant slight differences. H. erectus and earlier hominins seem to have had growth patterns that are possibly more similar to the one in chimpanzees than to the modern human pattern but new high-quality data are required to substantiate this claim.
Brain Shape
Evolutionary Changes of Endocranial Shape
Research about hominin brain evolution has focused on brain size although the quality of data sometimes does not allow incontrovertible conclusions as the preceding discussion showed. Furthermore, endocranial surface features have been analyzed and interpreted as impressions of brain convolutions, i.e. gyri and sulci of the brain (see Holloway 2014). Since different convolutions of the brain have particular cytoarchitecture specific for certain brain functions (see Sherwood et al. 2008; Reyes and Sherwood 2014), such endocranial surface details have been used to discuss the reorganization of the brain that went along with, but that was partly dissociated from brain size increases during hominin evolution (Falk et al. 2000; Holloway et al. 2004). However, endocranial impressions of brain convolutions are sometimes difficult to locate. Different interpretations of the location, presence and absence of specific sulci and gyri led to a huge body of literature but often not to a consensus (e.g., Falk 1980b; Holloway 1981; Falk 1983; Holloway 1984; Falk 1985).
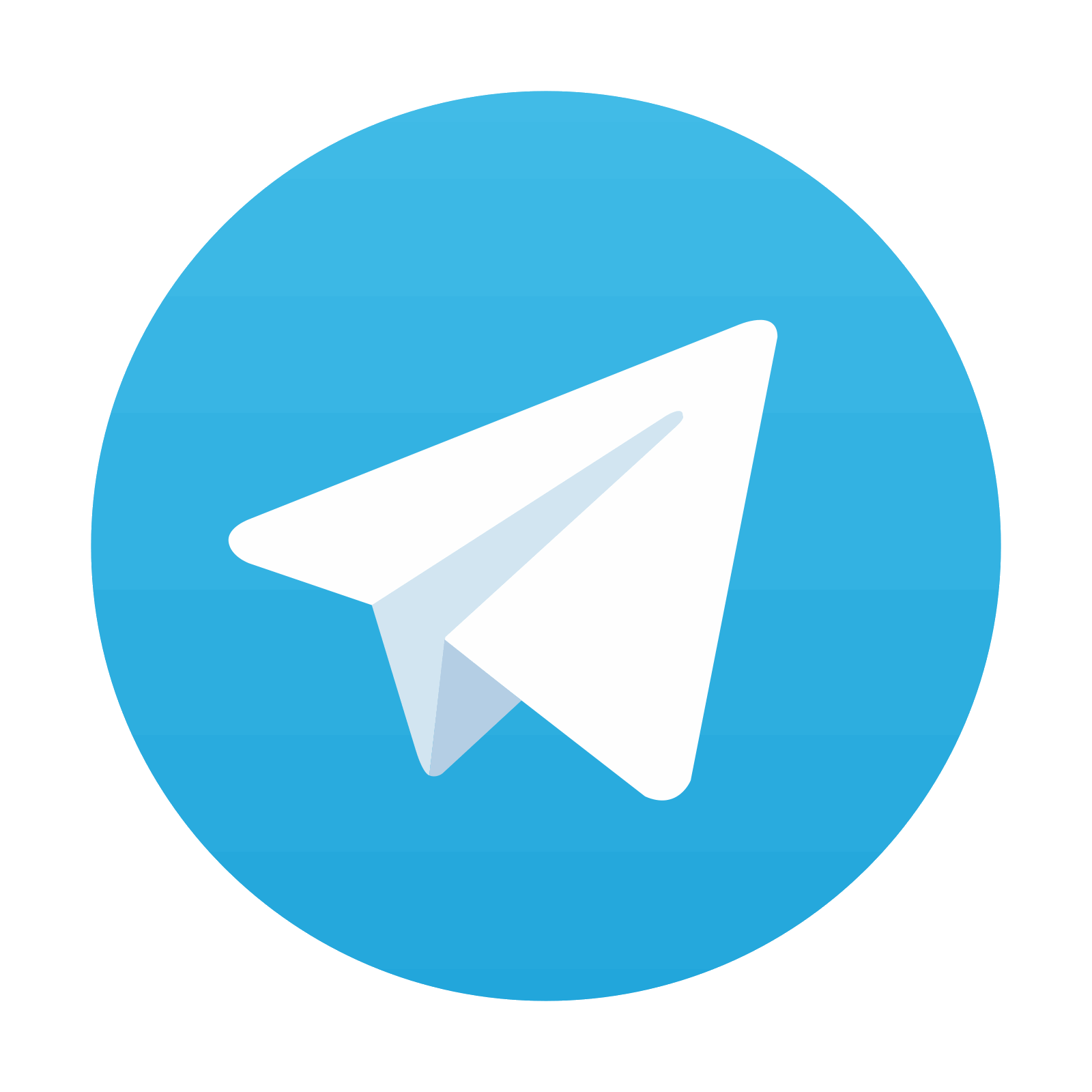
Stay updated, free articles. Join our Telegram channel
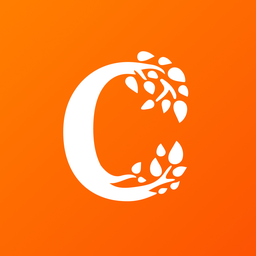
Full access? Get Clinical Tree
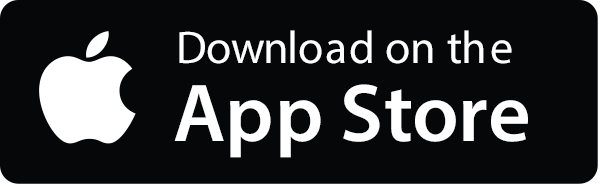
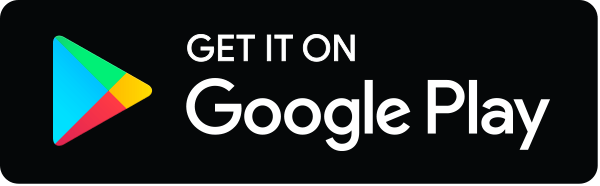