Fig. 6.1
Simulated data demonstrating how cross-sectional data (a) may not reveal an association (in this case positive) with time that is clearly seen with longitudinal data (b). Measurement values are simulated to represent a normal distribution with a positive disease duration effect in both cross-sectional and longitudinal data
6.3.2 Pathological Specificity of MRI Measures
Current MRI measures are not pathologically specific. They may be regarded as being weighted toward one process more than another, but they are not absolute measures. This is due to a combination of factors, some imaging and others tissue related.
First, the imaging methods themselves are tuned to produce scans with good contrast, high resolution, and limited artifacts, rather than quantifying a particular tissue characteristic. So, T2w scans are not usually described as T2 scans, because they are also influenced by other factors such as T1 relaxation times (RT), proton density (PD), scanner magnetic, and radiofrequency field homogeneity. There is a move within research to use MRI techniques that measure a particular feature and generate a quantitative image, so, for example, T2 RT maps can be produced that provide a measure of the T2 RT at a given point; in contrast, a T2w scan produces an image where the brightness of a point gives an indication of its relative, but not its actual, T2 RT.
Second, multiple pathological processes can affect the same MRI parameter. For example, T2 RT are related to axonal and myelin densities (sharing ∼60% variability) [29], although as axonal and myelin densities are also strongly correlated (also sharing ∼60% variability), it is not possible to determine if T2 RT are determined by axonal or myelin densities, or both.
6.4 The MRI Toolkit
MRI relies on the properties of atoms in magnetic fields and how they react when disturbed by electromagnetic (radiofrequency) pulses. While most images obtained in clinical practice are based on the signal from hydrogen in water, using proton spectroscopy, other molecules can be assessed, for example N-acetyl-aspartate which is found mostly in neurons and their axonal projections [30]. It is also possible to use other atoms as the basis of MRI, such as phosphorus and sodium, but due to their much lower abundance in tissues, the signal they produce is far weaker than that from protons in water, and the resultant images therefore have much lower signal to noise.
There are a variety of MRI methods available to image the brain and spinal cord. These may be broadly divided into four categories: macrostructural, that is those that generate anatomical images and allow localization of lesions, or enable tissue volumes to be measured; microstructural, that is those that yield measures of cellular physical features, such as the degree of myelination, or density of axons; molecular, which assess the amount a given molecule within a tissue, for example glutamate and glutamine, or lactate; and metabolic (including functional MRI), which assess features such as tissue blood flow and oxygen extraction. There has been some cross-sectional work demonstrating functional MRI [31–34] and perfusion [35–37] abnormalities in progressive MS, but longitudinal MRI or clinical follow-up studies have not been undertaken, and so the dynamics and prognostic significance of these measures is unknown. As such, it is not possible to determine their role in predicting or monitoring progressive MS, and we will not consider them further in this chapter.
6.4.1 Conventional Structural Scans
Conventional MRI scans generate anatomical images, and are tuned to maximize tissue contrast and make abnormalities obvious. In the case of brain and spinal cord imaging in MS, routine clinical and research scans include T1w and T2w images, and fluid-attenuated inversion recovery (FLAIR, which only provides useable image in the brain, not the spinal cord) sequences which suppress signal from CSF (Fig. 6.2). Lesions can be counted and their volume measured. Lesion counts are usually achieved manually, while lesion volumes are most often measured using a semi-automated lesion contouring method, for example [38].


Fig. 6.2
Matched axial slices through the cerebrum on T1-weighted (a), T2-weighted (b), and FLAIR scans (c). While MS lesions are more conspicuous on FLAIR, they are usually also seen on T2-weighted scans. In contrast, not all lesions seen on the FLAIR or T2-weighted scan are visible on the T1-weighted images
In the cerebral hemispheres, hyperintense WM lesions are more visible on FLAIR than T2w scans [39], while only a subset of lesions seen on T2w images are also apparent as hypointensities on T1w images (e.g., [40]). Interestingly, lesions seen on T1w scans may be more destructive than those observed on T2w scans alone [41], although correlations with EDSS scores are not markedly different [42]. Gadolinium (Gd) contrast can be given intravenously to demonstrate areas where there is breakdown in the blood–brain barrier, and on monthly scans identifies about twice as many active (new or enlarging) lesions than does FLAIR or T2w imaging [39]. There is some uncertainty as to the frequency of Gd-enhancing lesions in PPMS when compared with RRMS and SPMS, as both disease subtype and disease duration may be relevant (discussed below).
GM is extensively demyelinated in MS, but until recently, it has proven very difficult to see this using MRI. Conventional MRI techniques such as PD/T2w and FLAIR imaging are able to detect the majority of WM lesions (about 67% prospectively), but only a minority of GM cortical and deep lesions (about 13%) [43]. This considerable difference has led to a search for other MRI methods optimized to image GM lesions, with double inversion recovery (DIR) currently (as of May 2012) the most widely accepted technique. DIR is similar to FLAIR, suppressing signal from CSF, but in addition nulling signal from the WM, leaving only GM visible. GM lesions appear bright on such a scan (Fig. 6.3). However, while improving on FLAIR, DIR appears to detect only about a 40% more GM lesions [44], implying that it still does not identify the majority of such plaques. Despite this, GM lesions are still usually seen in people with SPMS, with higher cortical lesion loads found in people with SPMS when compared with RRMS (mean 11.3 compared with 5.4) [45]. We discuss this further below.


Fig. 6.3
Matched axial slices through the cerebrum on FLAIR (a) and DIR (b). While some cortical lesions are seen on FLAIR, more are detected on DIR. The open arrow marks a cortical lesion seen on both scans, the full arrows lesions clearly seen on DIR, but less readily so on FLAIR
6.4.2 Atrophy Measures
Measures of brain and spinal cord volumes have proven of great interest in MS as they appear to be more closely related to long-term disability than WM lesion loads [4, 5].
Techniques for assessing brain atrophy can be divided in to those that measure tissue volumes and those that directly assess change without first estimating volumes. Both approaches rely on high-resolution structural imaging (usually a 3D and T1-weighted scan with 1 mm3 resolution). Several brain segmentation techniques have been developed, including SIENA and SIENAX [46], SPM [47, 48], and FreeSurfer [49]. Brain volumes are frequently assessed as fractions of total intracranial volume, usually defined as the sum of GM, WM, and CSF. Such measures are less vulnerable to the effects of scanner scaling drift over time, with intracranial volume acting as a subject-specific reference. Scaling to intracranial volume also reduces intersubject variability, which in cross-sectional work allows more subtle disease effects to be observed when compared with absolute tissue volumes [23]. Change measures rely on warping one scan to match another, and measuring the amount of distortion required to achieve this [46, 50]. A significant issue in MS brain atrophy measurement is the effect WM lesions have on segmentation. On T1-weighted volumetric images, lesions appear darker than normal-appearing WM, and may be classified as GM or CSF instead. In addition, their presence may subtly distort segmentation of GM and WM boundaries throughout the brain, by making WM appear darker than it truly is. These effects can both be significantly reduced by filling WM lesions with simulated normal-appearing WM [51], although at present this requires time-consuming manual or semi-automated contouring of the lesions on volumetric scans.
Spinal cord atrophy is usually assessed by measuring cord cross-sectional area rather than volume. There are several reasons for this. First, there is marked intersubject variability in cord segmental lengths, but less noticeable variation in cross-sectional area [52], which means that intersubject variability in segmental volumes will be greater than segmental areas. Second, and more importantly, it is difficult to consistently identify spinal cord segmental levels using MRI, as there are few cord landmarks conspicuous enough to allow this. In comparison, it is relatively easy to locate the boundary between CSF and cord to measure cross-sectional area. Until recently, the most frequently used technique to achieve this relied on semi-automated contouring of a series of axial T1-weighted slices through the cervical cord (centred on C2/3) [53]. More recently, a nearly fully automated method has been developed to shrink wrap the cord in a computer-defined mesh, and from this extract measures of cross-sectional area [54, 55]. This active surface method is not only less user dependent, it also appears to be more reproducible than the established semi-automated technique, and this should translate into greater sensitivity to disease effects.
6.4.3 Microstructural Imaging
There are currently two main MRI techniques that assess tissue microstructure, magnetization transfer imaging (MTI), and diffusion tensor imaging (DTI).
MTI uses an indirect method to image protons associated closely with cell membranes. MTI usually takes the form of a magnetization transfer ratio (MTR) scan, generated from paired T2w scans, one of which has been prepared with a magnetization transfer pulse that excites bound protons such as those associated with cell membranes. The difference between the paired images provides a measure of the density of bound protons, and so an indication of the density of cell membranes. MTI is of particular interest in MS because it has been found to correlate with myelination [56], albeit with the caveat that it may also be significantly influenced by axonal density (the independence of this is difficult to determine as axonal density and myelination are also correlated [29]).
DTI uses the molecular movement of water in a tissue to determine structural characteristics. It relies upon the principle that water will diffuse more rapidly along cell membranes than through them. A DTI dataset comprises multiple images sensitized to water movement in many directions, and from this, information about the direction and extent of water movement at a given location can be extracted. Asymmetry of water diffusion is often measured as fractional anisotropy (FA), that is the proportion of molecular water movement along rather than across the main diffusion axis. The magnitude of water diffusion, regardless of direction, is estimated as the mean diffusivity (MD). FA is relatively higher in WM than GM, while MD may be marginally higher in GM than WM (e.g., [57]), and this reflects underlying tissue architectures: in WM tracts, with their highly ordered myelinated tracts, water diffusion will tend to occur along their axis; in contrast, the layered and columnar arrangement of neurons and their dendritic arbor will result in a more complicated diffusion profile, with relatively less directionally consistent diffusion.
6.4.4 Spectroscopy
Spectroscopy is perhaps the most challenging of the methods described here. It requires careful calibration of a scanner, and given the low concentrations of metabolites when compared with water, obtains data with low signal to noise. As such, it is only possible to obtain reliable measurements in large regions (cm3 compared with mm3 for the imaging methods outlined above). However, it enables the measurement of brain molecule concentrations in vivo, as such offers a unique perspective on pathology. It relies upon each molecule having a unique fingerprint that can be identified by looking at an MR spectrum (Fig. 6.4). Spectroscopy is not confined to molecules containing protons alone, for example phosphorous spectroscopy is also possible, but to date work in progressive MS has focussed on proton spectra.


Fig. 6.4
A proton spectrum from a single. PRESS voxel acquired with an echo time of 35 ms and repetition time of 3 s. Overlying this (solid black line) are the fitted metabolite peaks from LCModel [91]. The main peak at about 2.0 ppm is N-acetyl-aspartate (found mostly in neurons and their axons), at 3.0 ppm creatine and 3.2 ppm choline (Courtesy of Dr. Bhavana Solanky)
Only some molecules are present at sufficiently high concentrations to be able to discern spectroscopically. The main peaks visible on proton spectra are creatine and phosphocreatine (Cr), choline-containing compounds (Cho), N-acetyl-aspartate (NAA), myoinositol (Ins), and glutamate with glutamine. Of these, Cr is often used as an internal standard, with other molecular concentrations presented as a ratio to it, that is Cho/Cr or NAA/Cr ratios. However, this assumes that Cr is unaffected by disease, which may not be the case in MS, and as such there is a move toward absolute quantification of metabolite concentrations, that is measures in mmol/l. Of the metabolites mentioned, two are of particular interest as they appear to be relatively cell-specific: NAA, found mostly in neurons and their axonal projections [58], and Ins present in astroglia [58, 59].
6.5 Observing Progression Using MRI
6.5.1 Lesions
While new and contrast-enhancing lesions are seen in progressive MS, particularly in SPMS when compared with PPMS, they appear to be less clinically relevant than in RRMS.
Before looking at this further, it is worth flagging the caveat that estimates of lesion load change vary quite widely between studies even in the same subtype of MS. Taking SPMS as an example: over 1 year, Dalton et al. estimated that, on average, there was a 1.0 ml increase in T2w lesion load in 23 people with SPMS (and a 0.7 ml increase in 41 people with RRMS, using data from the placebo arm of a natalizumab trial) [60]; while Ge et al. [61] estimated a median decrease of 0.02 ml over a year in nine people with SPMS (and 0.35 ml increase in 27 people with RRMS); and Kapoor et al. observed an average yearly increase of 3.6 ml per year in 57 people with SPMS (data from the placebo arm of a trial of lamotrigine) [28]. From this, it can be seen than factors other than the disease subtype may be important determinants of lesion accrual.
First, comparing progressive MS with RRMS, the transition from RRMS to SPMS appears to be marked by a deceleration in lesion formation. Fisher et al. [17], in a 4-year follow-up study observed an average 1.34 ml increase in T2 lesion load in people with RRMS, 1.06 ml in those in transition to SPMS during this time, and 0.64 ml in people with established SPMS. Interestingly, there was a less clear-cut negative gradient for T1 lesions, with mean changes over 4 years of 0.84, 1.88, and 1.01 ml, respectively, perhaps hinting at a shift toward lower but more destructive inflammatory activity, or maturation within lesions that have already formed. These observations contrast most starkly with brain atrophy that seems to accelerate, particularly in GM, from RR through to SPMS (discussed further below).
Turning to differences in new T2-lesion formation in PP and SPMS, Kidd et al. [20] followed up a group of ten people with PPMS and nine with SPMS over 1 year. They found that while change in expanded disability status scale (EDSS) [11] was similar in both groups (about 1 EDSS point), there were noticeably more new brain T2 lesions in SPMS compared with PPMS patients (median 12.4 and 2.0, respectively). Interestingly, in this study, 95% of the lesions seen in people with SPMS enhanced, compared with 70% in PPMS, although the latter was almost entirely attributable to a single very active PPMS patient, and as such may not be typical (discussed further below). Changes in lesions loads were not found to correlate with change in EDSS, although this is perhaps not surprising given the small cohort and limited progression observed over the year. Over 2 years, lesion accrual in 56 people with SPMS was also not found to correlate with EDSS change: when comparing those who clinically progressed (17 subjects, mean EDSS change ∼1) with those who did not, increases in lesion load did not significantly differ between groups (4.8 ml vs. 6.4 ml, respectively) [62]. Similarly, in 41 PPMS patients followed up for 5 years, no associations between T2 lesion accumulation and increasing EDSS were seen, although the increase in disability was small (median 0.5 of an EDSS point), as was lesion accrual (about 1 ml per year) [63].
Considering contrast enhancement with Gd, an early serial study comparing people with established SPMS and PPMS (mean disease duration 7.8 and 7.0 years, respectively) found that over 6 months, enhancing lesions were much more common in SPMS than PPMS patients (mean about 18 new lesions per SPMS patient per year, of which ∼80% enhanced, compared with about three new lesions per PPMS patient per year, of which ∼5% enhanced) [64]. Similarly, Silver et al. [65] found that Gd-enhancement was more frequent in SPMS (∼57%, mean disease duration 12 years) than in PPMS (∼13%, 9 years). The enhancing lesion rates were found to be similar in another study of people with SPMS (60 patients, enhancing lesions at baseline in 53% and over 3 months new enhancing lesions in 70%) [66]. However, in a study of people with early PPMS (mean disease duration 3.3 years from clinical onset) using triple-dose Gd dosage, enhancing lesions appeared to be more frequent (∼42%) [67]. Overall, this suggests that contrast enhancement is relatively common in SPMS, less frequent in PPMS, but that in PPMS at least it may be more noticeable earlier rather than later in the course of the disease.
DIR, used to assess GM lesions, has only become established within the last 5 years, and so there has been very little work comparing subtypes of MS. In a study of 31 people with SPMS and 76 with RRMS [45], using DIR to identify new cortical lesions over 3 years, GM lesion formation appeared to be higher in people with SPMS compared with RRMS (0.6 ml compared with 0.3 ml over 3 years), in contrast to T2w (mostly WM) lesion formation which was marginally greater in RRMS compared with SPMS (1.6 and 1.3 ml, respectively). A significant association between GM lesion formation and increasing EDSS was noted in the whole group. This suggests that there is greater GM lesion formation in SPMS compared with RRMS, and that GM lesion may play a role in clinical progression, at least in SPMS. A direct comparison between SPMS and PPMS has not been undertaken, but they also seem to be common in PPMS. Calabrese et al. [68] noted cortical lesions in about 80% of the early PPMS cohort (mean disease duration 4 years) they studied, with an average cortical lesion load of 6.4 lesions per patient, and over 2 years, nearly 60% of the group accrued at least one new cortical lesion (averaging about 0.8 per patient per year).
Overall, these studies suggest that WM lesion formation has limited use as a marker of progression in progressive MS, but they also flag a potential role for GM lesions.
6.5.2 Atrophy
CNS tissue volume loss is emerging as a key marker of clinically relevant pathology in MS. It is thought mostly to reflect neurodegeneration, albeit with qualification that loss of other cell types can also lead to volume loss [69]. Early work sought to measure ventricular or whole brain volumes, but with the advent of technology allowing separate GM and WM volume estimates, it appears that progressive brain atrophy occurs predominantly in the GM [17].
Due to the complexities of segmenting brain tissues, early work simplified this by measuring distances between structures, such a ventricular width, or cross-sectional areas of the brain over a limited number of slices [23]. One such technique estimated ventricular volumes by contouring them on axial slices through the brain [60], and using this technique, Dalton et al. demonstrated greater ventricular enlargement over a year in people with SPMS compared with RRMS (mean increase 1.1 ml in 23 people with SPMS and 0.5 ml in 41 with RRMS). Similarly, progressive ventricular enlargement has been observed in PPMS: in the placebo arm of an exploratory trial of interferon in PPMS [70], over 2 years, in 20 people, ventricular volumes were observed to increase by 2.8 ml, which, per year, is close to the rate noted for SPMS. Ventricular enlargement has been found to correlate with whole brain volumes (r = −0.65 in a mixed cohort of 86 people with MS (22 with RRMS, 32 with PP and SPMS)) [71].
Whole brain atrophy has also been seen in progressive subtypes of MS, but in most recent serial studies has been measured alongside tissue-specific GM and WM volume estimates. In PPMS, Sastre-Garriga et al. [72] found in a group of 31 people with early PPMS (within 5 years of symptom onset), ∼1% decrease in brain parenchymal fractional (BPF; the total volume of brain tissue divided by total intracranial volume) over 1 year, but a ∼1.5% reduction in GM fraction (GMF) and nonsignificant change in WM fraction (WMF). Tissue volume changes did not correlate with changes in EDSS or MSFC scores. BPF correlated with baseline PD-weighted and contrast-enhancing lesion loads, albeit it modestly (r = −0.36 and −0.42, respectively), and the latter appeared to be driven by subtle associations with WMF rather than GMF. Similar results have been obtained in SPMS, with a 2-year follow-up study of 56 patients revealing ∼0.6% reduction in whole brain volume, this being mostly attributable to GM (∼1.2%) rather than WM (∼0.1%) loss [62]. While WM atrophy did not correlate with change in EDSS, whole brain and GM atrophy and spinal cord area did correlate with MSFC scores (r = 0.35, 0.42, and 0.34, respectively). Calabrese et al., in their study of cortical lesions in RRMS and SPMS, also measured tissue volumes, and observed ∼1.5% reduction in GMF in SPMS, compared with ∼1.2% in RRMS, which was found to be significantly different [45]. In the serial study undertaken by Fisher et al. [17] (discussed above), in the same cohort, they noted a clear acceleration of GMF but not WMF loss: in healthy controls, GMF declined by 0.03% per year, in RRMS 0.23%, those converting to SPMS 0.35%, and those with established SPMS 0.39%; in contrast, the corresponding figures for WMF were 0.08%, 0.24%, 0.33%, and 0.25%, respectively. They also observed greater GMF but not WMF loss associated with increased EDSS scores, but did not explore this relationship in the RR and SP subgroups separately. In the SPMS group, baseline contrast-enhancing lesion loads, lesion, and normal-appearing brain tissue MTR did not predict subsequent brain atrophy, while in RRMS they did. This suggests that lesion formation is a less important factor determining GM atrophy in SPMS when compared with RRMS. There is also evidence suggesting that GM atrophy is not evenly distributed throughout GM in progressive MS. Sepulcre and colleagues [73], studying the same PPMS cohort as Sastre-Garriga et al. [72], observed, over 1 year, preferential loss in deep GM and some cortical areas.
There has been a recent resurgence in interest in spinal cord atrophy with the recognition that it may have an important, and at least partly independent, role to play in determining long-term disability [5, 6]. Earlier work had already shown that it was clinically relevant, but there have been very few serial studies. Stevenson et al. [74] observed progressive cervical cord atrophy in people with progressive MS. Over 1 year, mean upper cervical cord area decreased in 12 people with PPMS by −3.52 mm2, compared with −0.26 mm2 in 6 people with SPMS (although due caution should be exercised given the small sample size). In a larger group including 6 people with RR and four with benign MS, they did not find an association between change in EDSS and cord atrophy, although only 8 of the 28 people studied were found to have progressed over this period. More recently, Agosta et al. [75] reported that cervical cord area decreased by 3.1 mm2 (4%) in 15 people with PPMS over a mean of 2.4 years, compared with 2.2 ml2 in 14 people with SPMS (3%) and 5.4 mm2 in 13 people with RRMS (7%), but did not undertake comparisons between groups. That cord atrophy is at least semi-independent of brain volume loss, has been noted in mixed cohorts [5, 6], and the absence of a correlation between cord area and partial brain volume in PPMS reinforces this [63].
Considered together, these longitudinal studies suggest that in progressive MS, brain atrophy occurs more rapidly in GM than WM volume. Tissue-specific atrophy rates in PPMS and SPMS have not been systematically compared, but it does appear that brain atrophy is at least as rapid in PPMS as in SPMS. In contrast to lesion load, brain and spinal cord atrophy have been found to be more consistently associated with clinical progression. Spinal cord atrophy also occurs, at least semi-independently of brain atrophy: while the studies are small, they too suggest that cord atrophy is at least as fast in PPMS when compared with SPMS.
6.5.3 Microstructural and Metabolic Studies
There has been very little serial work using MTR, DTI, or proton spectroscopy in progressive MS.
Considering first MT imaging, in an early study looking at whole brain and normal-appearing MTR values, changes over 1 year were apparent in SPMS but not PPMS or RRMS [76]. While the RRMS cohort was quite large (39 subjects), the progressive cohorts were smaller (19 SPMS and 9 PPMS). Subsequently, in the placebo arm of an SPMS interferon trial, a decrease in whole brain MTR was seen over 3 years, confirming the previous observation, although this did not correlate with EDSS [77]. More recent work in early PPMS (within 5 years of symptom onset) has found more rapid changes in GM rather than WM. Khaleeli et al. [78] studied 30 people with PPMS, following them up clinically and radiologically over 1 year. Fifteen healthy controls were also scanned. They observed a greater reduction in mean MTR in normal-appearing GM (NAGM; mean 0.9% in the year) in patients compared with controls, but no significant difference in normal-appearing WM (NAWM; 0.6% in the year). They did not report on associations between change in clinical and MTR measures, but did note that baseline mean NAWM MTR predicted change in EDSS and MSFC over the subsequent year. They also found that changes in MTR values correlated with atrophy, although sharing less than 50% variability.
Turning to DTI, a longitudinal assessment of this has been undertaken by Agosta et al. in a mixed cohort of people with MS (13 RR, 14 SP, and 15 PP) [75], followed up on average for 2.4 years. In this work, cervical cord area was noted to decline, and cervical cord mean diffusivity (MD) increased and FA decreased. Concurrent with this, brain NAWM MD was observed to increase, NAWM FA declined (only in people with SPMS), but brain GM MD did not change. Cord and brain DTI, and cord atrophy measures did not correlate with change in EDSS scores, although progression over the year was limited in all the MS subgroups (on average between 0.5 and 1 EDSS points), limiting the scope to detect associations. Interestingly, in a study evaluating the predictive value of DTI in PPMS, Rovaris et al. [79] found that in a multivariate analysis, GM MD, but not NAWM MD or FA, was an independent predictor of clinical deterioration over the next 5 years, suggesting that even if diffusion changes are less dynamic in GM than WM, they are of greater clinical consequence.
Proton spectroscopy has been used in one serial PPMS study of glatiramer acetate. [80] studied 37 people with PPMS (nine in the placebo arm) over 1 year, and 19 people over 3 years. The work was undertaken at four centers, and this was found to have a significant effect in metabolite ratios. They estimated NAA/Cr and Cho/Cr ratios, and noted no change over time, and no difference between people receiving the active agent or placebo.
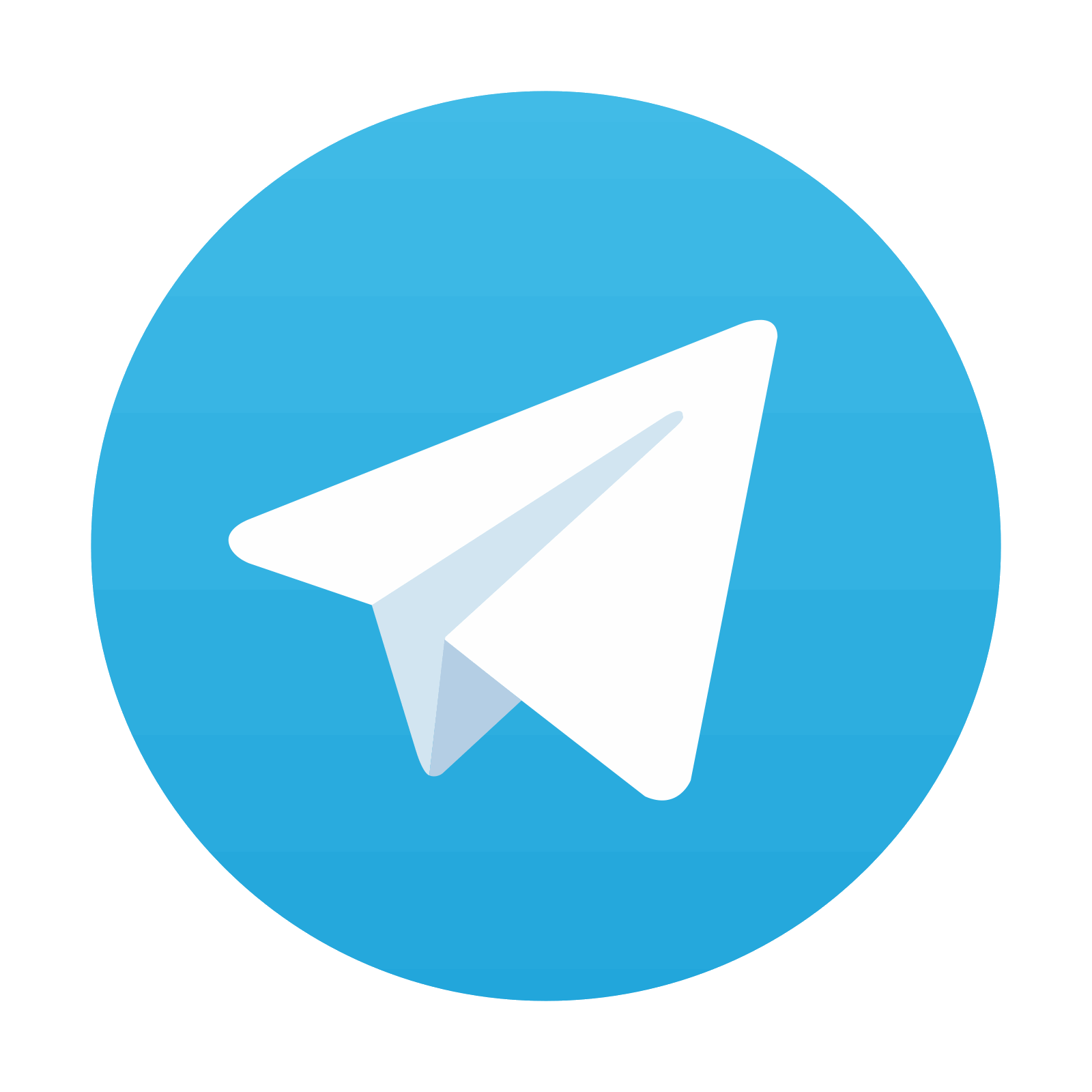
Stay updated, free articles. Join our Telegram channel
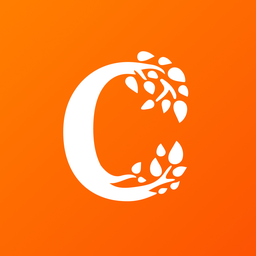
Full access? Get Clinical Tree
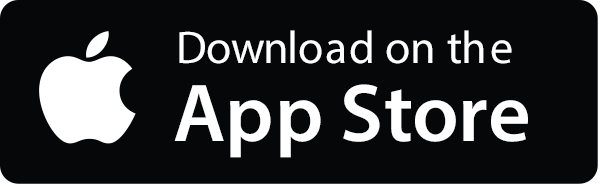
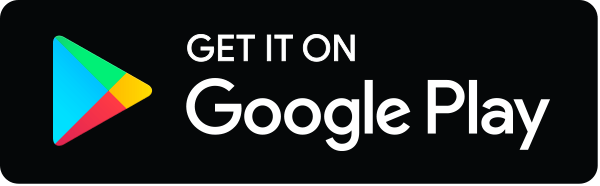