Imaging plays a major role in the identification, grading, and treatment of cerebral arteriovenous malformations and cerebral dural arteriovenous fistulas. Digital subtraction angiography is the gold standard in the diagnosis and characterization of these vascular malformations, but advances in both magnetic resonance imaging and computed tomography, including advanced imaging techniques, have provided new tools for further characterizing these lesions as well as the surrounding brain structures that may be affected. This article discusses the role of conventional as well as advanced imaging modalities that are providing novel ways to characterize these vascular malformations.
Imaging plays a major role in the identification, grading, and treatment of cerebral arteriovenous malformations (AVMs) and cerebral dural arteriovenous fistulas (DAVFs). Digital subtraction angiography (DSA) is the gold standard in the diagnosis and characterization of these vascular malformations, but advances in both magnetic resonance imaging (MRI) and computed tomography (CT), including advanced imaging techniques, have provided new tools for further characterizing these lesions as well as the surrounding brain structures that may be affected. Advances in MRI techniques have also allowed better noninvasive characterization of these vascular lesions in ways that previously could only be possible with catheter angiography. In this article, the authors discuss the role of conventional as well as advanced imaging modalities that are providing novel ways to characterize these vascular malformations.
Cerebral arteriovenous malformations
General Features
Cerebral AVMs represent congenital vascular abnormalities, in which single or multiple arterial feeding vessels supply a dysplastic plexiform network of vessels (also known as the nidus) with direct shunting into the venous system. The nidus, which represents a conglomerate of dysplastic, thin-walled vessels, is histologically best characterized as a cluster of arterialized veins. The nidus can be supplied by one vascular distribution, or there can be supply from multiple vascular territories, representing a so-called borderzone AVM. Cerebral AVMs are thought to represent congenital lesions caused by alterations in the vascular modeling process, a theory supported by the vascular complexity of the lesions. There is little to no functional brain tissue within the AVM nidus, leading to the assumption that functional brain is displaced to the margins of the lesion.
Micro-AVMs are defined as lesions with a nidus that measures 1 cm or less. These lesions have historically been known as cryptic or occult AVMs because they were not readily seen on imaging studies previously. Nearly 40% to 50% of cerebral AVMs in the setting of hereditary hemorrhagic telangiectasia and 7% of sporadic AVMs are micro-AVMs. These lesions typically have a single normal-sized arterial feeder and a single draining vein. Micro-AVMs are typically located superficially, within the cortex or within the subarachnoid space.
Aneurysms are seen in up to 58% of AVMs. Flow-related aneurysms can be found on feeding arteries or within the AVM nidus, which can frequently serve as the focus of AVM rupture. Aneurysms can also occur on arteries remote from the AVM arterial supply. There can be significant varicosity and aneurysmal dilatation of draining venous structures, especially in the setting of high-flow AVM, which also increases the risk of hemorrhage. Direct arteriovenous connections may be present within the lesion and can generally only be identified on superselective microcatheter angiography. Flow-induced angiopathy is often associated and reflective of reactive endothelial hyperplasia; this can result in arterial or venous stenoses. Venous drainage can be superficial, deep, or a combination of the two. The drainage pathway is important to note on imaging studies because of the important role this factor plays in prognostication and in determining the treatment plan.
Risk of Hemorrhage
The overall estimated likelihood of AVM hemorrhage is between 2% and 4% per year, but can be as low as 0.9% per year without any associated risk factors. There is an associated 5% to 17.6% chance of death and 30% to 50% chance of permanent neurologic deficits in the setting of AVM hemorrhage. Many factors have been associated with an increased risk of AVM hemorrhage, including small AVM size, high-flow lesions with increased arterial and venous pressure, deep as well as infratentorial locations, and a history of previous AVM hemorrhage. In addition, other angiographic characteristics such as intranidal aneurysm(s), flow-related arterial aneurysms, presence of deep venous drainage, and venous stenosis also correlate with increased hemorrhagic risk. The importance of risk factors is, however, somewhat controversial. Duong and colleagues indicate that the most important risk factors for AVM hemorrhage are high arterial input pressure and exclusive deep venous drainage. During a prospective follow-up of 622 patients with AVM for a mean time period of 2.3 years, Stapf and colleagues found that the most important risk factors for hemorrhage of AVM are exclusive deep venous drainage, deep brain location, and history of previous AVM rupture. After an initial hemorrhagic event there is an increased risk of hemorrhage, ranging between 4.5% and 34.4%. There was an overall 6% rate of hemorrhage in patients who had experienced a previous hemorrhagic event, as compared with 1.3% for patients who did not have a history of AVM rupture. Borderzone location for an AVM, with supply from multiple arterial distributions, appears to be protective against hemorrhage.
Conventional Imaging: MRI and CT
CT and MRI are the typical initial imaging examinations performed on patients with AVMs, as the most common presentations are not specific for AVM and include intracranial hemorrhage, seizure, headache, and focal neurologic deficit. Large AVMs can be readily identified on CT and MRI, but smaller lesions may be more difficult to detect. Noncontrast head CT is usually the initial imaging examination based on the clinical presentation, to evaluate for any hemorrhage. On noncontrast head CT examination, prominent serpentine hyperattenuating structures ( Fig. 1 A, B), representing draining veins, components of the nidus, or dilated arterial feeders, suggest a diagnosis of AVM. Curvilinear or speckled calcifications involving the nidus or the feeding or draining vessels can also be seen. The AVM typically does not cause much mass effect, unless there has been hemorrhage. Instead, there may be hypoattenuation and volume loss in the brain parenchyma surrounding the nidus, relating to gliosis or hemosiderin deposition from previous hemorrhage. Hemorrhage at presentation is seen in 42% to 72% of cases, most commonly parenchymal, followed by subarachnoid and intraventricular hemorrhage.

In the absence of a known underlying etiology for parenchymal hemorrhage, CT angiography is frequently the follow-up examination to evaluate for a possible underlying lesion, such as AVM or ruptured aneurysm. CT angiography (CTA) will show the enhancing nidus as well as prominent draining veins, but evaluation is limited on conventional CTA, as temporal flow-related changes are not evaluated. Characteristic features of the AVM, such as feeding arterial and draining venous stenosis, flow-related arterial and intranidal aneurysms, and draining venous aneurysms, may be seen, but are not as well depicted or evaluated as on DSA. Significant venous contamination, if present, limits differentiation between draining veins and normal veins. Even on an excellent quality CTA study, small and micro-AVMs may be difficult to detect. In the setting of hemorrhage, the nidus may be compressed by the hematoma, limiting detection of a small underlying lesion. However, this applies to all imaging modalities, including CTA, MRI/MR angiography (MRA), and even DSA. In the setting of a prominent parenchymal hematoma, it is advised that repeat imaging be performed 4 to 6 weeks after detection of the hematoma. This interval allows time for mass effect related to the hemorrhage to decrease, sometimes permitting for identification of the responsible lesion.
On conventional MRI, dilated arterial feeding arteries, the nidus, and draining veins are represented as flow voids ( Fig. 1 C, D). T2 and FLAIR (fluid-attenuated inversion recovery) hyperintensity involving the adjacent brain parenchyma frequently relates to gliosis (see Fig. 1 C, D), which is a result of hypoperfusion of portions of the surrounding brain secondary to cerebrovascular steal. There can be T1 and T2 hypointensity and susceptibility on b0 sequences in the adjacent brain structures, relating to hemosiderin from previous hemorrhage. MRI can depict important details of mass effect on adjacent brain structures or involvement of eloquent cortex, which cannot be readily shown on DSA. On postcontrast T1-weighted sequences, there will frequently be enhancement of portions of the AVM nidus. With small AVMs, a small area of cortical and subcortical enhancement may be the only finding on conventional imaging ( Fig. 2 ).

MRI in conjunction with MRA can be a useful noninvasive imaging tool in the postradiosurgery setting to evaluate for residual AVM, even though DSA remains the gold standard. The residual nidus is represented as residual flow voids, with persistent feeding arteries and draining veins. On postcontrast sequences, evaluation for AVM-related enhancement is limited, as postradiosurgery patients can frequently have radiation-induced parenchymal enhancement. There was 80% sensitivity (64/80) and 100% specificity (84/84) on conventional MRI for residual AVM postradiosurgery when compared with DSA, according to Pollock and colleagues.
Susceptibility-Weighted Imaging
Susceptibility-weighted imaging (SWI) relies on exquisite sensitivity to magnetic susceptibility effects. Sensitivity to magnetic susceptibility is optimized by using long echo time high-resolution flow-compensated 3-dimensional (3D) gradient echo sequences with filtered phase information. This technique relies on the paramagnetic characteristics of deoxyhemoglobin and the resultant phase differences between venous blood and the surrounding brain parenchyma, as well as arterial blood. SWI is very sensitive for the depiction of venous structures as well as extravascular blood.
SWI can provide helpful information in the evaluation of venous structures draining AVMs. Frequently on conventional imaging and time-of-flight (TOF) MRA, differentiation between arterial feeders and draining veins can be difficult. SWI more accurately shows draining venous structures than TOF MRA and conventional MRI. High-flow arteriovenous shunting in AVM can be accurately depicted on SWI as a hyperintense venous signal, which correlates well with findings of arteriovenous shunting on DSA ( Fig. 1 F). This finding relates to rapid wash-in of high-flow oxygenated blood into draining veins. SWI is accurate in the evaluation of residual arteriovenous shunting after AVM treatment; in the posttreatment setting, residual ectatic veins will have decreased signal due to deoxyhemoglobin, whereas persistent arteriovenous shunting will show a hyperintense venous signal. SWI is advantageous to TOF MR venography because it is more sensitive to slow flow within draining venous structures, and there is no loss of signal in vessels coursing in the imaging plane. SWI can be helpful in depicting micro-AVMs, which may not be depicted on conventional MRI or TOF MRA, by accurately portraying small draining venous structures otherwise not seen. Early identification of micro-AVMs is important, as these lesions have an increased risk of hemorrhage.
Time-Resolved MRA
Time-resolved MRA is an evolving technique that is showing early promise in its ability to characterize cerebral AVMs. DSA currently remains the gold standard in evaluating the angioarchitecture of cerebral AVMs because of its combination of exquisite spatial and temporal resolution. However, DSA is invasive, expensive, exposes the patient and the operator to ionizing radiation, and has a small but finite risk of associated symptomatic ischemic events. There have been significant advances in contrast-enhanced time-resolved MRA sequences, which allow image acquisition rates of up to 3 frames per second. 3-T MRI units are favored over 1.5-T units because they provide increased signal-to-noise ratio, permitting the use of undersampling techniques, which increases temporal resolution.
Time-resolved MRA can show flow of contrast through each component of the AVM, confirming the suspected findings on conventional MRI/TOF MRA and CT/CTA examinations. Time-resolved contrast-enhanced MRA has been shown to correlate well with DSA in terms of AVM grading, nidus size and location evaluation, venous drainage pattern, and arterial feeders in multiple studies. MRA sequences of high spatial resolution and high temporal resolution can be performed as separate sequences during a study to achieve both improved spatial and temporal resolution. At present, however, DSA remains the gold standard in AVM evaluation because at this point, time-resolved MRA cannot match the high spatial and temporal resolution of DSA.
Time-of-Flight MRA
3D TOF MRA without contrast has remained a first-line diagnostic tool in the evaluation of cerebrovascular disease, including cerebral aneurysms and steno-occlusive disease. TOF MRA is frequently one of the first examinations obtained when there is an initial diagnosis of cerebral AVM, in addition to conventional MRI (see Fig. 1 ). TOF MRA does not typically rely on gadolinium contrast agents, is easy to perform, and provides high-resolution images. The background stationary soft tissues within a slab are saturated with radiofrequency (RF) pulses on TOF imaging. Inflowing blood maintains its signal and appears bright, representing flow-related enhancement.
Contrast-enhanced 3D TOF MRA, when performed in conjunction with conventional MRI, can provide valuable pretreatment information and an overall broad characterization of AVM. While DSA can be used for evaluation of the AVM prior to radiosurgery, contrast-enhanced 3D TOF MRA might provide a more accurate means of delineating the 3D contour and size of the AVM in the pretreatment setting. Accurate delineation of the AVM nidus is important for adequate AVM coverage, which is the most important factor for successful treatment. Overcoverage can lead to unnecessary irradiation of adjacent brain tissue, and undercoverage will result in inadequate AVM treatment. Because DSA is a 2-dimensional (2D) image, the true nidal size can be obscured or misinterpreted, due to overlapping adjacent vascular structures or inhomogeneous enhancement ; this can lead to overestimation of the nidus size and inaccurate delineation of nidal contours. Contrast-enhanced 3D TOF MRA can also be a useful tool in the evaluation for postradiotherapy residual AVM. 3D TOF MRA with contrast, as compared with DSA, detected nearly all cases of residual AVM but its specificity was 68% and diagnostic accuracy only 77%, according to Lee and colleagues. The false-positive cases were related to residual ectatic vessels around the thrombosed nidus in combination with radiation-induced parenchymal enhancement.
Unenhanced 3D TOF MRA is limited in the evaluation of feeding arteries and draining veins when compared with DSA. Only 65% of arterial feeders detected on DSA can be seen on 3-T 3D TOF MRA, and only 71% of superficial and 60% of deep draining veins are seen on 3T 3D TOF MRA in comparison with DSA. These percentages are even lower on 1.5-T scans. In addition, it can be difficult to differentiate between arterial feeders and draining veins, especially with complex lesions with multiple feeding arteries and draining veins. Limited visualization of draining veins in part relates to the application of a saturation band, which is applied to suppress venous flow. TOF MRA is limited in its evaluation of turbulent, complex flow, which is frequently seen in AVMs. The turbulent blood flow is saturated by multiple RF pulses. Another limitation of 3D TOF MRA is the evaluation of small AVMs, as frequently the nidus, feeding arteries, and draining veins may not be seen. Contrast-enhanced TOF MRA has overcome many of the artifacts that are seen with unenhanced TOF MRA, including loss of flow-related enhancement due to slow, turbulent, or complex flow.
Diffusion Tensor Imaging
Diffusion tensor imaging relies on directional diffusion (anisotropy) of water molecules along nerve axons and nerve bundles. In normal white matter tracts, myelin sheaths and axonal cell membranes restrict water diffusion in the direction perpendicular to the nerve fibers. The primary direction of water motion is parallel to the orientation of the white matter tract. Tensor maps can be used to generate 3D tractogram maps, which represent the trajectory of the white matter tract.
Diffusion tensor imaging can serve as a valuable noninvasive preoperative and preradiosurgery tool to evaluate for involvement and proximity of cerebral AVMs to white matter tracts. Accurate assessment of white matter tracts can be performed in the setting of adjacent AVMs with or without hemorrhage ( Fig. 3 B, C). The proximity of the AVM to eloquent white matter tracts may eliminate surgery as a treatment option, due to the risk of white matter tract injury intraoperatively or secondary to hemorrhage. Diffusion tensor tractography can be useful in determining the radiation dose to the corticospinal tract prior to stereotactic radiosurgery, and thus can help in therapeutic planning, allowing for delivery of an effective dose to the nidus while minimizing the risk of posttreatment complications.

Diffusion tensor imaging and tractography of AVMs can depict fiber tract displacement or disruption, or decreased fiber tract density relating to involvement. Some reports have indicated that there is a strong correlation between involvement of the corticospinal tract by hemorrhage or edema from AVM and contralateral hemiparesis, whereas others have not been able to find an association. In the authors’ experience with diffusion tensor imaging, there may be the appearance of displacement, compression, or involvement of eloquent white matter tracts, without associated clinical deficits.
There are a few limitations of diffusion tensor imaging and tractography that are important. Hemorrhage or calcifications related to cerebral AVM, surgical clips, or postoperative changes can cause susceptibility artifacts on diffusion tensor imaging, which are problematic because the echo planar imaging technique is typically used. In the setting of edema or involvement of a white matter tract, a drop in fractional anisotropy (FA) values may fall below the preset threshold for tractography, leading to the false-positive appearance of disruption of this fiber tract. The FA threshold can be lowered to allow for detection through low-anisotropy regions; however, this may allow for the appearance of spurious white matter tracts. The authors prefer to rely on the color FA maps to evaluate for eloquent white matter tract involvement or displacement, due to the aforementioned limitations of tractography. At their institution the authors typically perform diffusion tensor imaging in conjunction with task-based functional MRI for preoperative planning, mostly in the setting of brain tumors, but occasionally in the setting of AVMs (see Fig. 3 ).
Functional MRI in the Assessment of Cerebral Arteriovenous Malformation
Blood oxygen level–dependent (BOLD) functional MRI is a noninvasive technique that has developed into an important tool for preoperative planning. This technique relies on changes of susceptibility in regions of brain activated by specific tasks, related to slight changes of blood oxygenation. With neuronal activation there is a change in the microenvironment, leading to vascular dilatation, and an increase in blood oxygenation. Functional MRI allows for eloquent cortical mapping (both lateralization and localization) and evaluation of the spatial relationship of eloquent brain to the pathologic lesions that are being evaluated preoperatively. Frequently structures such as the motor or sensory strips, Broca’s area, or Wernicke’s area can be displaced, distorted, or involved by pathologic processes such as AVMs. In addition, normal variations of cortical surface anatomy make localization difficult or even impossible without functional imaging. Functional imaging allows for prediction of resectability of a lesion, and identification of the components of a lesion that can be removed without sacrificing eloquent brain structures. Preoperative functional MRI can shorten the surgical time as well as alter the surgical approach. With preoperative functional MRI smaller craniotomies are needed, as limited intraoperative cortical mapping can be used. Negative cortical mapping can be performed with electrical stimulation of the margins of the lesions to evaluate for any eloquent cortex.
Functional MRI can serve as a helpful planning tool in the treatment of AVMs. Damage to eloquent cortex can be avoided before embolization, surgical resection, or radiosurgery of these lesions. Preoperative functional MRI may also alter the clinical plan, from surgery to radiosurgery, depending on the anatomic relationship to eloquent cortex. 51 When considering that AVMs represent congenital lesions, it is no surprise that intrahemispheric and interhemispheric cortical reorganization can be seen in such patients. An important limitation of task-based functional MRI in the evaluation of AVMs, as well as tumors, is neurovascular uncoupling. Functional MRI relies on cerebrovascular dilatation related to increased neuronal activity, leading to increased blood oxygen content. In the setting of cerebrovascular abnormality, such as is seen with AVM and dysplastic vessels supplying tumors, the abnormal vessels do not respond to these stimuli, and there can be diminished or no task-related BOLD activation of normal functioning neurons within the lesion or in the adjacent cortex. Neurovascular uncoupling can simulate cortical reorganization, as the normal cortex will have no task-related BOLD activation (see Fig. 3 D, E). At their institution, the authors use breath-hold BOLD cerebrovascular reactivity (CVR) maps to qualitatively evaluate for neurovascular uncoupling. Abnormal vessels such as those seen within and around cerebral AVM will not vasodilate in response to increased carbon dioxide levels, and will show absent or diminished activity on the CVR map relative to the rest of the brain.
MRI Perfusion
First-pass dynamic susceptibility-weighted contrast-enhanced (DSC) MR perfusion imaging is an established perfusion technique used to evaluate relative cerebral blood volume (rCBV), mean transit time (MTT), relative cerebral blood flow (rCBF), and time to peak (TTP). The major clinical use of perfusion imaging is to evaluate ischemic penumbra in the setting of acute stroke and for tumor evaluation.
On MR perfusion examinations in the setting of cerebral AVM, there is increased CBF and CBV across the lesion, as expected, relating to increased transnidal flow. Perfusion of perinidal and remote brain relative to the AVM in the ipsilateral hemisphere is variable. Guo and colleagues reported two dominant patterns of perfusion, both of which showed increased perinidal rCBF and rCBV; this may result from dilatation of the perinidal arteries, which may be adaptive in response to adjacent low-resistance AVM feeding arterial structures, or could relate to angiogenesis in response to hypoperfusion secondary to cerebral steal. A third pattern, however, is also discussed, in which there is reduced perinidal rCBV and rCBF, secondary to cerebral steal by the adjacent AVM. After radiosurgery treatment, rCBV and rCBF values of the perinidal and remote brain parenchyma trended toward the normal values of the contralateral brain.
Digital Subtraction Angiography
Despite advancements in noninvasive imaging modalities, conventional DSA remains the gold standard for evaluation of cerebrovascular disease, including cerebral AVMs. The combination of exceptional spatial (0.2 mm) and temporal resolution (up to 24 frames per second) is unmatched by other imaging modalities. DSA is optimal for the evaluation of AVM angioarchitecture, including improved detection of associated intranidal aneurysms, obstruction of venous outflow, arterial feeder anatomy, and venous drainage patterns ( Fig. 4 ). Superselective microcatheter angiography can help further characterize AVM angioarchitecture by allowing targeting of specific feeding arterial branches. DSA is the optimal imaging modality to evaluate for potential risk factors for AVM rupture, including deep venous drainage, venous outflow stenosis or occlusion, intranidal and arterial feeder aneurysms, and high-flow lesions with increased arterial and venous pressure. DSA can be used for preradiosurgery delineation of the AVM nidus. Catheter angiography allows for planning of endovascular embolization, which is frequently an important component of the treatment strategy.

DSA is the gold standard for preradiosurgery evaluation of nidus size due to its excellent spatial and temporal resolution, allowing for evaluation of the size of the nidus before there is venous filling. However, because DSA is a 2D image depicting a 3D structure, the true nidal size can be inadequately assessed by overlap from adjacent vascular structures or inhomogeneous enhancement. As described later, 3D rotational angiography supplements 2D DSA for the evaluation of the 3D contours of the nidus.
Limitations of DSA include that it is a 2D modality evaluating a 3D structure. Dilated venous varicosities and arterial feeders will overlap with the nidus on 2D DSA, obscuring evaluation of nidal size and contours. 3D rotational DSA (3D RDSA) provides 3D images that use the high spatial resolution of conventional DSA. This technique can provide improved evaluation of the relationship between the nidus, feeding arteries, and venous output. There is better depiction of the 3D contours of the nidus shape, allowing for superior determination of the radiation target.
Because DSA does not show the relationship and involvement of the adjacent brain parenchyma, conventional imaging modalities are frequently used for this evaluation. With the advent of cone-beam CT technology, some of these limitations of DSA can be overcome. DSA is an invasive procedure, with a small but finite risk of permanent neurologic deficit of approximately 0.1% to 1.0%. Moreover, there are procedural risks associated with exposure to ionizing radiation and iodinated contrast dyes.
C-Arm Cone-Beam CT
C-arm cone-beam CT (CBCT) represents a 3D C-arm mounted flat-panel detector cone-beam CT system, which produces high-resolution volumetric data acquisition with a single rotation of the C-arm. The system is incorporated into the interventional suite, and high-resolution multiplanar reformats can be quickly generated at an independent workstation. After selecting the vascular structures of interest by catheter-directed interventional procedure, diluted contrast is injected immediately before imaging, thus providing high-resolution, selective cross-sectional 3D images, focusing on the vascular pathology of interest.
The applications of this technology are extensive, including evaluation of intracranial and extracranial vascular abnormalities, including AVMs, DAVFs, and aneurysms. CBCT has also shown the ability to show exquisite detail regarding venous intracranial anatomy, which cannot be achieved with MRA, CTA, or DSA. During intracranial vascular embolization, CBCT can help guide management in the setting of complications by producing high-quality CT scans.
In the setting of cerebral AVM, CBCT provides excellent high-resolution 3D cross-sectional images, allowing for better characterization of the vascular lesion. Feeding arterial and draining venous structures can be better depicted, with cross-sectional images providing the exact point of communication with the nidus. Nidal angioarchitecture can also be better determined. CBCT can provide improved localization and characterization of nidal and flow-related venous and arterial aneurysms (see Fig. 4 E, F). The relationship of the vascular malformation to adjacent brain structures is better depicted on CBCT than on 2D DSA contrast injections alone. Gupta and colleagues reported a case in which CBCT allowed for better characterization of diffuse basal ganglionic AVM arterial supply, allowing for improved targeted embolization while sparing vascular supply to the intervening internal capsule.
Dural arteriovenous fistulas
General Features
DAVFs make up 10% to 15% of intracranial vascular malformations, and are abnormal connections between dural arteries and dural venous sinuses, meningeal veins, or cortical veins. The etiopathogenesis remains to be fully elucidated, but DAVFs have been associated with history of trauma, previous craniotomy, or dural venous sinus thrombosis. In the setting of interrupted normal sinus outflow and elevated local venous pressures, it is hypothesized that tiny physiologic shunts may enlarge, or neoangiogenic factors may promote the formation of pathologic connections. Adult DAVFs, which constitute the majority of lesions, are most common at the transverse, sigmoid, and cavernous sinuses. There is also a subset of pediatric lesions that can often involve the torcula, superior sagittal sinus, and large venous lakes. The etiology of pediatric DAVFs is thought to be congenital or related to birth trauma, infection, or in utero venous thrombosis.
Adult intracranial DAVFs present most commonly in the fifth and sixth decades of life. Pulsatile tinnitus is one of the most common symptoms of transverse and sigmoid sinus lesions, and is related to increased flow through the dural venous sinuses. Cavernous sinus DAVFs can cause ophthalmoplegia, proptosis, chemosis, retroorbital pain, and decreased visual acuity. Additional nonhemorrhagic neurologic deficits (NHND) include seizures, dementia, chronic headache, and cranial nerve abnormalities. Hemorrhage is more common in high-grade lesions, which demonstrate retrograde cortical venous drainage (CVD).
Accurate diagnosis and classification of DAVFs is essential in identifying patients with high-risk lesions and to triage them toward appropriate therapies. The Borden and Cognard classification schemes, which have been well substantiated as predictors of DAVF behavior, both emphasize the relationship between CVD and increased risk of hemorrhage or NHND. The absence of CVD (Borden I; Cognard IIa) is associated with a benign natural history. These patients typically present with pulsatile tinnitus and other signs of increased dural venous drainage, but have a low risk of hemorrhage. The presence of CVD (Borden II, III; Cognard IIb–V) indicates a more aggressive lesion, associated with an annual mortality rate of up to 10.4%, hemorrhage rate of up to 8.1%, and risk of NHND of up to 6.9%. Zipfel and colleagues have further subclassified lesions exhibiting CVD into those that present with hemorrhage or NHND and those that are detected incidentally or present with nonaggressive symptoms (ie, tinnitus, ophthalmologic symptoms). The former group has a higher annual risk of hemorrhage at approximately 7.5% whereas the latter group has lower risk at approximately 1.5%, suggesting that this additional subclassification may improve the accuracy of risk stratification in DAVF patients.
The diagnosis of DAVFs at an early stage may be difficult because of nonspecific clinical and imaging findings. A high index of suspicion is thus necessary when a patient presents with an unexplained constellation of neurologic complaints and deficits. The goal of imaging is not only to determine the presence or absence of a DAVF but also to clarify the pattern of venous drainage. Once the morphology and hemodynamics of the lesion are appropriately delineated, the need to pursue aggressive therapies can be determined.
Conventional Cross-Sectional Imaging
Although the diagnosis of DAVFs has traditionally been by DSA, many of these lesions are now first detected or suspected on cross-sectional imaging. Noncontrast head CT is often used as an initial screening tool to determine the presence of hemorrhage or edema from venous congestion, but is otherwise limited in its diagnostic contribution. MRI can demonstrate engorged vessels, dilated venous pouches, or abnormal vascular enhancement in the presence of a DAVF. Several of these findings are the result of venous hypertension or retrograde CVD, leading to medullary venous congestion. In a series of 27 patients, Kwon and colleagues demonstrated a significant association between dilated vessels or prominent vascular enhancement with the presence of retrograde CVD. Venous hypertension in high-grade lesions is also thought to contribute to abnormalities such as white matter T2 hyperintensity, intracranial hemorrhage, or venous infarction. Low-grade lesions may exhibit only flow-void clustering, engorged veins, or proptosis. The presence of any suspicious findings on MRI should warrant further imaging evaluation with dynamic CTA, MRA, or DSA for clarification of lesion grade and management decision making.
MRI Perfusion
DSC MR perfusion can be a very sensitive marker for the evaluation of impaired venous drainage in the setting of cerebral DAVF, and permits for the quantitative evaluation of MTT, CBV, and CBF. In patients with cerebral DAVF with retrograde CVD, there are increased CBV values involving the affected cerebral hemisphere secondary to impaired venous drainage. The correlation of increased parenchymal perfusion to retrograde cortical venous drainage is controversial. According to Noguchi and colleagues, CBV ratios (CBV values of the affected hemisphere relative to the contralateral normal hemisphere) show increased values in the affected cerebral hemisphere with DAVF and retrograde CVD, in comparison with normal control subjects and patients with DAVF without retrograde CVD. CBV ratios in patients with DAVF without retrograde CVD approximated ratios in control subjects. Fujita and colleagues claim that in the setting of DAVF with or without retrograde CVD, there is increased CBV ratio, prolonged transit time, and decreased CBF in the affected cerebral hemisphere, consistent with a pattern-impaired venous outflow. No statistically significant difference was found in perfusion between DAVF with or without retrograde cortical venous drainage. After treatment of DAVF with retrograde CVD, CBV ratios of the affected cerebral hemispheres decreased, trending toward the values seen in normal control subjects.
Susceptibility-Weighted Imaging
SWI is a technique that allows for improved detection of extravascular blood and better characterization of veins carrying deoxygenated blood. SWI can clearly depict arteriovenous shunting in cerebral DAVF, represented as hyperintense venous signal relating to rapid wash-in of oxygenated blood.
SWI can accurately depict retrograde CVD associated with DAVF. In the setting of DAVF there is impaired venous drainage and increased venous pressure, resulting in cortical venous engorgement, as well as prolonged venous stagnation, leading to increased venous oxygen extraction. These factors are thought to result in increased prominence of cortical veins on SWI in the setting of retrograde CVD with DAVF. According to Noguchi and colleagues, SWI depicts dilated superficial veins involving the ipsilateral cerebral hemisphere in all cases of DAVF with retrograde CVD, whereas conventional MRI sequences depict dilated superficial veins in only 71% of cases. SWI portrays prominent deep draining veins in 64% of the affected cerebral hemispheres, whereas T2-weighted sequences show prominent veins in only 34% of cases. This finding corresponds to the expected increased rCBV values on DSC perfusion sequences of the affected cerebral hemisphere, secondary to impaired venous drainage.
MR Angiography
3D TOF MRA is limited in the evaluation of DAVFs because of the lack of hemodynamic information, but can suggest the presence of a lesion by abnormal flow enhancement. In a series of 11 patients by Kwon and colleagues, MRA demonstrated fistula-site enhancement in 83% of cases, and venous flow-related enhancement in 91% of cases. Fistula-site enhancement was detected only in cases with CVD; this reliance in flow dynamics contributed to the failure in visualization of one DAVF (8%) with slow venous flow. Lesions with smaller and multiple arterial feeders and draining veins, as commonly seen in carotid-cavernous fistulas, are also more difficult to visualize precisely. The dependence of TOF MRA on flow velocity can present a challenge of distinguishing abnormal, fast, or slow shunt flow from normal flow in certain cases.
Dynamic MRA using time-resolved imaging of contrast kinetics (TRICKS) has evolved substantially in recent years and has developed an increasing role in DAVF imaging. The dynamic resolution of this modality is adequate for separating early arterial, arterial, parenchymal, and venous phases. Initial studies using 1.5-T scanners were limited by low spatial resolution, signal-to-noise ratio, and region of coverage, precluding clear identification of feeding arteries and draining veins or the presence of CVD. The advent of 3-T scanning technology has offered a solution to many of these issues, and recent studies on whole-head time-resolved MRA (trMRA) have suggested the advantages of this modality as a screening and surveillance tool. In a retrospective series of 42 patients (20 of whom harbored DAVFs), comparing the results of trMRA to DSA for presence, location, and classification of DAVFs, Farb and colleagues reported only a 2.4% error rate of diagnosis using trMRA. These errors included 2 cases in which CVD was interpreted on trMRA images, but not demonstrated on DSA, and one case of a Borden I lesion that was missed on trMRA. Nishimura and colleagues reported a prospective series of 18 patients in which DSA and trMRA results were independently interpreted by radiologists who were blinded to the results of the other modality. Intermodality agreement for fistula site and lesion grade, based on venous drainage pattern, was 100%, but for the identification of arterial feeders was 89%. These data reflected the limitations of trMRA spatial resolution compared with DSA (1 × 1 × 1.5 mm vs submillimeter, respectively), which hinders its ability to fully characterize lesions for therapeutic purposes. Despite this limitation, the investigators suggest that trMRA is a suitable modality for primary diagnosis and follow-up evaluations for DAVFs. It must be emphasized, however, that in patients who demonstrate objective clinical signs of a vascular lesion, DSA should still be performed to definitively rule out a DAVF, irrespective of negative MRA findings.
CT Angiography
CTA can provide a useful adjunct for treatment planning by defining the location of the arteriovenous shunt relative to surrounding brain and skull anatomy. CTA can be performed rapidly, is widely available, and can be easily combined with other CT evaluations. DAVFs, however, can be obscured by overlapping osseous structures on conventional CTA, reducing the sensitivity of the modality to as low as 15.4% in some studies. Several techniques for bone subtraction, including direct subtraction, local subtraction, matched mask bone elimination, and hybrid CTA, have been investigated as potential solutions to these limitations. Hybrid CTA uses reconstruction algorithms to remove bone structures while preserving the appearance of vascular channels, and has demonstrated promising results in recent studies. Lee and colleagues analyzed the diagnostic efficacy of 64-detector-row hybrid CTA in the evaluation of 24 DAVFs, using DSA as the reference standard. The most common findings on CTA were asymmetric sinus enhancement (92%), engorged arteries (79%), and enhancing transosseous vessels (79%). Lesion grade by CTA was confirmed by DSA in 100% of cavernous sinus lesions and 78% to 89% of transverse-sigmoid lesions. Hybrid CTA images were also suitable for treatment planning in most cases. Hybrid CTA lacks dynamic information, and requires further experience before its full role in DAVF evaluation can be determined.
The advent of 320-detector-row CT scanners has also opened investigations into 4-dimensional (4D) dynamic CTA for the evaluation of DAVFs ( Fig. 5 ). The appeal of this technology is similar to that of trMRA, as a potential tool to replace DSA for primary diagnosis and follow-up in patients who would otherwise require repeat DSA studies. Willems and colleagues have described their preliminary experience with 4D CTA in the evaluation of 11 DAVFs. DSA and CTA studies were interpreted independently by two blinded observers using a standardized scoring system. Ten of 11 cases were successfully detected on 4D CTA and agreed fully with the Borden classification on DSA. However, one Borden III lesion (9%), which had presented with a hemorrhage, was undetected on 4D CTA as a result of slow flow and low shunt volume. Large arterial feeders were recognized in 6 of 10 cases on 4D CTA, but additional contributors were missed in up to 8 of 10 cases on 4D CTA. The investigators concluded that 4D CTA currently lacks the spatial resolution to sufficiently delineate the angioarchitecture of DAVFs, and suggested the continued use of DSA for diagnosis and treatment planning. Of interest, the spatial and temporal resolution achieved on 4D CTA is greater than that of trMRA, but studies have demonstrated superior sensitivity for DAVF detection with trMRA. With continued developments in CT scanning technologies and reconstruction algorithms, 4D CTA will undoubtedly continue to advance, and further evaluation of its diagnostic value as an adjunct to DSA is merited. As with any CT-based technology, consideration of radiation dose will also ultimately be important in determining the optimal application of this modality toward patient care.
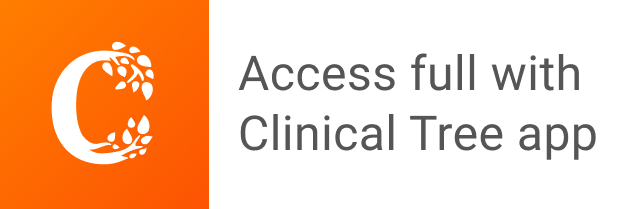