of the world’s population, have some form of disability, with rates increasing due to population ageing [17].
This has lead over the last years to an increasing interest in understanding the mechanisms of function recovery. This interest is fundamental also because of the enormous variety of impairments that may affect the recovery of functionality, thus life in society.
2 Neurorehabilitation and the Neural System
For many years it was believed that in the adult the nervous system is almost fixed. This was found not to be true, so that now brain plasticity is recognized as a fundamental property of the nervous system.
According to Oxford Dictionaries [33], we can define plasticity as the quality of being easily shaped or moulded. Applying this definition to the neural field, we have that plasticity, in this case brain plasticity, is the capacity for continuous alteration of the neural pathways and synapses of the living brain and nervous system in response to experience or injury that involves the formation of new pathways and synapses and the elimination or modification of existing ones [32].
This means that the brain is constructed to change. In fact “Plasticity is defined as the brain’s capacity to be shaped by experience, its capacity to learn and remember and ability to reorganise and recover after injury” [23].
This is why moderate spontaneous functional recovery can be found in patients following neural injuries and what rehabilitation tries to work on, as without plasticity there wouldn’t be recovery but only a deficit compensation.
In fact a lesion has also effects on areas that are functionally connected with the lesion site. Brain damage is always a damage to the entire network, so that recovery happens mostly in remote regions.
The complete mechanisms of plasticity are not completely understood [22], but it is known that the brain is able to reorganize the neural pathways, both for learning new skills and recovering from injury.
This is true also for the spinal cord, where there is an activity-dependent plasticity.
As new learning changes the spinal cord, compensatory plasticity in brain and spinal cord preserves the old behaviours. Each behaviour repeatedly induces plasticity to preserve its key features despite the plasticity induced by other behaviours.
If an old behaviour is impaired, a new behaviour that targets beneficial plasticity to an important site may improve the old behaviour.
This capacity of compensatory plasticity preserves fundamental functionality like locomotion.
Therapy in neurorehabilitation should be directed to take advantage of the plasticity of the CNS by a functional training.
Training and exercise have effects on cellular and molecular function involved in plasticity.
For example one of the established training techniques following Spinal Cord Injury (SCI) is treadmill based gait training, that has been successfully translated from animal studies to clinic and that has been found to improve connectivity of spared corticospinal pathways [13].
2.1 Why Develop New Technologies for Neurorehabilitation?
The main approaches of neural engineering used to rehabilitate impaired motor function are:
1.
Restoration: retain existing structures, both anatomical and neural, and control them for re-establishing a motor function.
2.
Replacement: substitution of the impaired motor apparatus with an artificial one controlled by residual structures.
There are different motivations for developing new technologies for rehabilitation [61]:
Improved technology may allow more therapy with less supervision, reducing also the costs.
Thanks to the increasing possibility to measure and quantify functional recovery, therapy could be quantified more accurately and new diagnostic possibilities can arise.
This new technology has the potential to permit new types of therapy, for example continuous therapy with wearable devices.
Some kinds of technologies could allow to move rehabilitation to the patient’s home.
Better devices could substitute lost functions (e.g. amputated limbs) so that the user is less restricted in his daily life.
Nowadays a great variety of new technologies is increasingly taking part of the clinical practice, improving the potential of therapists and clinicians to diagnose and rehabilitate. Wearable robotics, virtual reality, brain-machine interfaces and neural prosthetics are playing a leading role in this innovating process [57], and follow the footprints of other devices like Cochlear Implants and Visual Prosthetics.
2.2 Limits of Neurorehabilitation
Following neurorehabilitation many patients with acquired brain injuries observe modest benefits and when progress is made the acquired gains may fail to be maintained outside the medical setting. One of the biggest problems is that rehabilitation strategies are quickly abandoned when active therapy has ceased, thus it is necessary to bring therapy in everyday life to create a major bond between rehabilitation and life after treatment [68] and therefore increase the amount of therapy a patient can receive.
This is particularly true when talking about upper limb rehabilitation, as the latter is often abandoned early because of the adoption of compensatory strategies and the fact that most daily activities can be performed with the intact limb [44]. Also, because of the fact that the one-handed task is more difficult, family members will end up doing certain activities for the patient, causing lack of training in everyday life.
Another problem is given by the high costs of maintaining the therapy at home and the fact that often the patient is abandoned at home with the equipment [25], without the necessary support to continue his rehabilitation.
New technologies, like mobile apps, gamification [21] and portable devices, can become a permanent and seamlessly transparent rehabilitation therapy after the treatment is finished and the patient is sent home. These are technologies that will enable people to treat themselves with very low professional intervention, and may motivate them to keep improving their situation long after the formal treatment. Also, they will become cheaper over time, allowing more and more people to use them.
Also techniques in telerehabilitation will have to ensure that machine-mediated therapies fit to the patient at a particular stage of recovery [25].
Obviously a match between the patient’s and the rehabilitation team’s goals is required, both in the medical setting and in home therapy. The prescription of the intervention has to be not only on the basis of knowledge of neurorehabilitation because often what a patient needs is not what he/she wants.
Greater progress may be achieved if the clinician is able to assist the patient in achieving his goals or making progress that can motivate him/her to further commitment.
This means also that the primary aim of the treatment should be directed to improve movement disorders and not the clinical signs.
3 Major Challenges to Face
There are several technologies and application areas of neurorehabilitation and neural engineering, so there are different specific challenges that have to be faced in the next years to allow widespread outside of the research field.
3.1 Robotic Rehabilitation
In rehabilitation, the goal of the different exercises is to perform specific movements to provoke motor plasticity and improve motor recovery, minimizing functional deficits.
Recovery of the motor function can occur through neural reorganization finding alternative paths to activating the muscles for a task, or using alternative muscles in compensatory strategies [53, 55].
As commonly stated, traditional physical therapy is hard work, both for the patient and the therapist (often more than one is needed, especially for gait rehabilitation), which may lead to injuries. Moreover the duration of the therapy session is often limited by the therapist’s endurance.
This is where robots come into hand.
The rapid growth in this field can be attributed to:
the emergence of hardware for haptics and advanced robotics, that permit to operate in a safe environment for the patient and the therapist.
the drop of computing costs and the emergence of software for real-time control.
Robotic rehabilitation aims to reduce the workload and the physical effort of the therapist, allowing more intensive and repetitive motions, and to assess quantitatively the motor recovery by measuring different variables, like force and movement patterns.
One of the main problems of robot-based neurorehabilitation is to get the new treatment accepted, remembering the fact that acceptance is a necessary but not sufficient condition for the technology adoption.
Surely the fact that rehabilitation robots can be used both for gaining information and for intervention could influence the adoption of this technology, but it is necessary to validate the ability of this information to measure underlying phenomena and to give a realistic quantitative measurement of the recovery process.
Rehabilitation robots are used for intervention on:
Lower limbs, where the training has to be repetitive and task-specific. The stimulus has also to be appropriately intense, as in locomotion the load is essential for appropriate leg muscle activation.
Upper limbs, where movement targets are acquired from a variety of sensory channels including vision and touch. This information is used to update internal models to encode also the sensory consequences of the interactions.
Tables 1 and 2 present a synthesis of some of the studies that have investigated the use of robot therapy, highlighting the prescription of the intervention and the Functional Scales used to measure the outcomes. It must be considered that although there are a number of smaller pilot studies, there are only few larger clinical trials evidencing the use of robot therapy [43].
Table 1
Clinical studies about rehabilitation using robot therapy for the upper limb
Device | Disability | Functional scale | Prescription |
---|---|---|---|
MIT-MANUS (Volpe, 2000) [69] | 2 weeks after single stroke | MPS, MSS, FIM | Regular therapy ![]() |
MIT-MANUS (Fasoli, 2003) [20] | Single, unilateral stroke within the past 1–5 years | AS, FMA of upper extremity function, MSS, Medical research council MPS | 4–5 h weekly for 4 weeks |
MIT-MANUS (Krebs, 1999) [37] | Hemiparesis | FMA for upper extremity function, MPS for shoulder and elbow, MSS for shoulder and elbow, MSS for wrist and fingers | Conventional therapy ![]() |
IMotion2 (MacClellan, 2005) [49] | Stroke, more than 6 months after stroke, shoulder and elbow deficits | Upper-limb MSS, Wolf motor function test, MPS, FMA for upper extremity function | 18 sessions over 3 weeks, two sessions a day at 1-h each, 3 days a week |
MIME—Stäubli PUMA-260 (Lum, 1999) [45] | Stroke, 1–45 months post-stroke | FMA, strength improvements in joint actions | 24 1-h sessions over 2 months |
MIME—PUMA-560 (Lum, 2002) [46] | Stroke, more than 6 months after cerebrovascular accident, chronic hemiparesis | FMA, FIM, biomechanic measures of strength and reaching kinematics | 24 1-h sessions over 2 months at least 6 months post first stroke |
MIME—PUMA-560 (Lum, 2006) [47] | Stroke, subacute | FMA for upper extremity function, MSS, FIM, MPS, mAS | 15 1-h treatment sessions over 4 weeks |
GENTLE/s [10] | Stroke, residual arm disfunction | FMA, MAS, mAS | 30 min of intervention three times per week |
Stroke, at least 6 months after brain injury | CMSA, Rancho functional test of the upper extremity | 24 1-h therapy sessions over 2 months | |
Robot-assisted arm trainer (Hesse, 2003) [29] | Stroke, more than 6 months after stroke, chronic hemiparesis | mAS score for spasticity, arm section of the Rivermead motor assessment score | 15 min net treatment with arm-trainer every workday for 3 weeks (15 sessions) ![]() |
Armeo spring (Colomer 2013) [9] | Stroke, chronic hemiparesis | mAS, motricity index, FMA, MAS, manual function test, Wolf motor function test | 3 one-h sessions per week for a total of 36 sessions |
Table 2
Clinical studies about rehabilitation using robot therapy for gait
Device | Disability | Functional scale | Prescription |
---|---|---|---|
Lokomat (Hidler, 2009) [30] | Stroke, less than 6 months | BBS, FAC, NIH stroke scale, MAS, Rivermead mobility index, FAI, SF-36 health survey | 45 min sessions, 3 days per week, for 8–10 weeks, for a maximum total of 24 sessions |
Lokomat (Hornby, 2008) [31] | Stroke, chronicity | Emory functional ambulation profile, BBS, FAI, Medical outcomes questionnaire (SF-36), mAS | 12 30-min sessions |
Lokomat (Husemann, 2007) [35] | Stroke, subacute | Massachussetts general hospital FAC, 10m time walking test, mAS, MI, German version of the BI | 30 min of conventional physiotherapy ![]() |
Lokomat (Mayr, 2007) [51] | Stroke, 0.5–10 months, inability to walk unaided | EU-walking scale, Rivermead motor assessment scale, MI, Medical research council scale of strength, AS of tone | group A: 3 weeks of Lokomat training, 3 weeks of conventional physical therapy, 3 weeks of Lokomat training. Group B: 3 weeks of conventional physical therapy, 3 weeks of Lokomat training, 3 weeks of conventional physical therapy. Treatment applied 5 times a week, for up to 30 min. |
Gait trainer (Pohl, 2007) [56] | Stroke, subacute | ADL, BI, FAC, Rivermead mobility index, MI | 20 min locomotor training ![]() |
Gait trainer (Werner, 2002) [70] | Stroke, subacute | FAC, Rivermead motor assessment, mAS | A: 2 weeks of gait trainer therapy, every workday, with a net walking time of 15–20 min. B: 2 weeks of treadmill therapy with partial body weight support, every workday, with a net walking time of 15–20 min. Group A: pattern ABA. Group B: pattern BAB |
Electromechanical gait trainer (Tong, 2006) [66] | Stroke, subacute | Elderly mobility scale, BBS, FAC, MI, FIM, BI | 5 sessions per week for 4 weeks, 20 min of gait training as part of 1.5 h of rehabilitation session |
These main fields of application influence the problems and challenges to be faced, even if there are some in common.
Although the field of rehabilitation robotics is strongly emerging and an increasing number of systems has entered the market over the last years or is in development and in trial in research institutes, a widespread use of these systems especially for personal use in home care and home rehabilitation has not occurred yet.
There are multiple reasons that limit the spread of those systems.
Especially the integration of robotic technology, like actuating systems and sensor systems, into rehabilitation devices strongly increases the costs of such devices.
Therefore less hospitals or even private users can afford such devices and insurance companies do not cover the costs.
Surely a key challenge is how to enhance the therapist’s skills with the robot technology, considering the robot as a tool. This means to use the robot to have the possibility to carry out longer and more intensive rehabilitation sessions without overloading the therapist, allowing the latter to focus more on the patient and its movements.
The usability of new systems can be limited, also due to the increased complexity of the devices. A specialized training is needed for the therapists to be able to use them and the setup time may also be too stressful for the patient. This can result in a limited acceptance from both the medical personnel and the patients.
This influences also the engineering evolution of this technology, as there is a need for more integrated solutions that ideally have similar protocols and interfaces, reducing the learning curve by the medical staff.
A further limit to overcome for the robots for upper limb rehabilitation is the division between reach and grasp investigating the integration in single devices, as the recovery of hand motor function is crucial to perform activities of daily living [52].
All kinds of rehabilitation robots have to be designed to assist “as needed”, because if the movement strategy is invariant, the neural control circuit becomes non-responsive. Ideally, the robot has to exert no interference unless the patient is unable to perform a task.
The goal must be to reduce the dependence on the robotic mechanisms to encourage relearning, using also strategies involving other kinds of technology (Sect. 3.2).
Despite the great possibilities offered by this type of technology rates of adoption in rehabilitation are very low.
This could be changed by getting robotic technologies past the feasibility study stage and analysing the economic sustainability, as cost is one of the big issues.
Current systems are often bulky while the mobile systems still lack long duration power supply solutions.
3.2 Virtual Rehabilitation
Virtual Reality is defined as “The computer-generated simulation of a three-dimensional image or environment that can be interacted with in a seemingly real or physical way by a person using special electronic equipment, such as a helmet with a screen inside or gloves fitted with sensors” [33].
The recent advances in this field, mostly driven by the entertainment industry, have led to an increasing availability of devices that can be used for rehabilitation purposes [28]. The availability of low cost HD displays and the possibility to use also everyday systems like smartphones as virtual reality devices has led to a reduction of hardware prices.
Virtual Reality allows a great influence on therapy when coupled with other methods: combined with rehabilitation robotics it allows to influence the feedback received by the patient through perturbation. Virtual systems can distort reality, thus trying to enhance improvements taking advantage of error augmentation and tricking the nervous system with altered sensory feedback [25].
One of the great advantages of Virtual Rehabilitation is interactivity and motivation [6]. This is especially true in game-based therapeutic approaches. The real-time multi-sensory feedback can be used for correction and the control over the different scenarios allows to achieve things impossible in the real world for the patient. Another great advantage is the real-time data collection and storage. Remote data access is fundamental for another field in which Virtual Reality can be useful, which is Virtual Telerehabilitation. Telerehabilitation may be beneficial for the reduction of healthcare costs. Since the goal is to bring the rehabilitation home with the patient, there is the need for mobile, networked treatments for long-term training, monitoring and online communication with the therapist. Despite the enormous potential in this field, there are great challenges to face for the widespread adoption of Virtual rehabilitation. The therapist’s attitude fearing substitution is a great gap to overcome. Also the interfaces present problems, as they were not designed as medical equipment: this often leads to the difficulty of sterilization for repeated use by different patients.
Even if costs have dropped significantly in the last years, the prices are still prohibitive for health clinics, also because here are almost no commercial systems available. This is also related to the fact that there is a great lack of cost-benefit analysis. The long-term consequences are not known and there are only few medical studies, so that the lack of outcome data for improvements does not satisfy the critics of the medical environment. Also compatibility between the different systems is a great issue.
Telerehabilitation has also additional challenges, due to the inadequate communication infrastructure that may limit videoconferencing with the patients in case of difficulty. Another aspect is the safety of the patient, as they may risk to re-injure themselves. Thus control softwares need to be integrated to make sure the patient is not exercising at higher level than prescribed or for longer than necessary [6]. While with telerehabilitation there is the risk of losing specificity and customizability of the treatment, it can be very useful if there is also remote control by the therapist, so that the medical staff can better structure the therapy, both for in-hospital and at-home sessions.
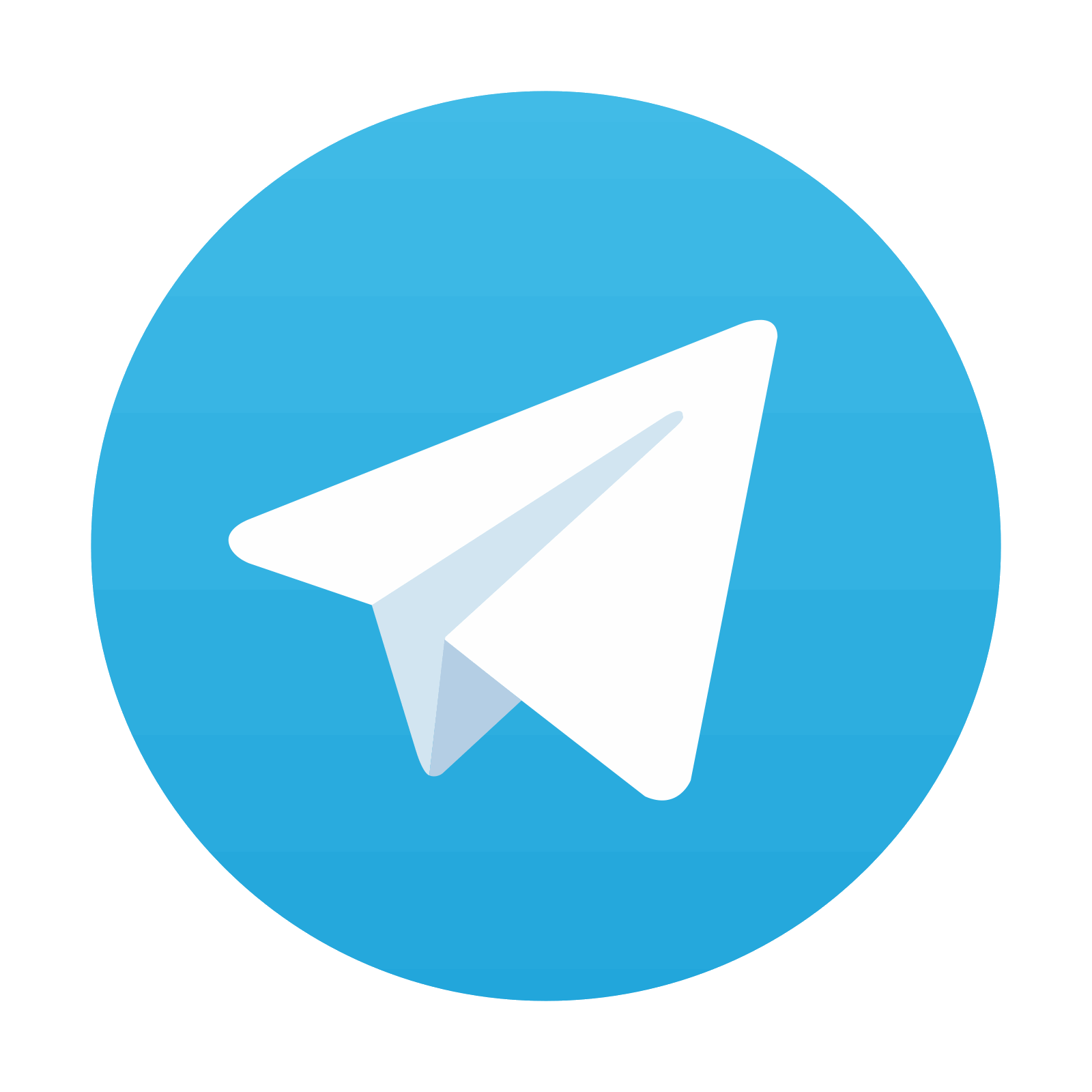
Stay updated, free articles. Join our Telegram channel
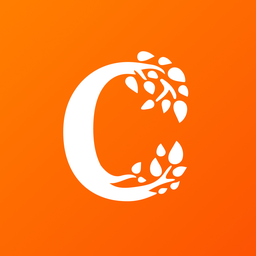
Full access? Get Clinical Tree
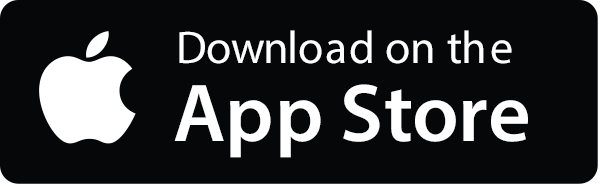
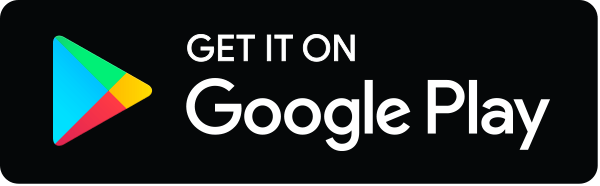