Ultrasounds
1794: Discovered by L. Spallanzani investigating bats’ movements in the dark
1842: Description of Doppler effect
1880: Curie brothers discovered the piezoelectric effect (US generation)
1915: After Titanic sinking in 1912, P. Langevin invented the first transducer which later led to the development of SONAR (SOund NAvigation and Ranging)
1947: First paper on the use of US for medical diagnosis of brain tumors by Dr. K. Dussik
1955: First echography by I. Edler
1980: Doppler echography
X-rays
1895: Discovered by W. C. Roentgen, Nobel Prize in 1901—first radiography (hand of his wife)
1913: W. Coolidge invented the X-ray tube
1972: First X-ray scanner or CT—computerized tomography by A. MacLeod Cormack and G. N. Hounsfield, Nobel Prize in 1979
MRI
1946: Description of nuclear magnetic resonance (NMR) phenomenon by F. Bloch and E. Purcell, Nobel Prize in 1952
During the 1950s and 1960s: development of NMR spectroscopy
1971: R. Damadian showed that NMR relaxation times of tissues and tumors differed, first MRI system “the indomitable MRI”
1973: First NMR image by P. C. Lauterbur
1975: R. Ernst introduced 2D NMR using phase and frequency encoding and the Fourier transform
1976: First human images by P. Mansfield and A. A. Maudsley
1990: Functional MRI by J. Belliveau
2003: Nobel Prize P. C. Lauterbur and P. Mansfield
Nuclear
1896: H. Becquerel discovered mysterious “rays” from uranium
1898: Discovery of natural radioactivity (polonium and radium) by M. and P. Curie
1903: H. Becquerel and M. and P. Curie received the Nobel Prize
1934: Discovery of artificially produced radioisotopes by I. and F. Joliot-Curie, Nobel Prize in 1935
1990: Development of nuclear medicine, with scintigraphy, single photon emission computed tomography (SPECT), and positron emission tomography (PET)
Small animals as rodents have been widely used for the development of animal models in biomedical and biological research. Mice are the commonly mammalian species used in research because of their rapid rate of reproduction, their short life cycle, their easy and low-cost husbandry, their known genetic background, and the possibility of genetic manipulation with transgenic and knockout techniques. However their small sizes (about 15–40 g) present severe challenges for in vivo imaging.
Clinical imaging equipments don’t allow investigating small animals as rodents mainly because of spatial resolution limitations. Indeed, mouse imaging requires a resolution inferior to 100 μm to visualize details comparable to clinical imaging. Many small-animal imaging devices have been adapted from clinical instrumentation (Table 2) [10, 11]. These high-resolution equipments developed in the past 30 years include micro-computed tomography (micro-CT) [12–21], magnetic resonance microscopy (MRM) [22, 23], ultrasound biomicroscopy (UBM) [24], micro-positron emission tomography (micro-PET) [25, 26], and high-resolution single photon emission computed tomography (SPECT) [27, 28]. Conversely, newer technologies such as optical imaging with fluorescent (FLI) and bioluminescent (BLI) tracer technology originated from in vitro cellular studies have been extended to animal applications [29]. Note that the term “micro” refers to the resolution scale; the physical background of these techniques applied in humans or animals is equivalent.
Table 2
Comparative spatial resolution of clinical and preclinical imaging modalities and associated technical refinements, inspired from [Small animal imaging: Basics and practical guide. Kiessling et al. 1st edition, 2011, springer ISBN 978-3-642-12944-5]
Modality | Spatial resolution | Human to animal technical refinements | |
---|---|---|---|
Clinical | Preclinical | ||
UBM | 1–2 mm | ≤100 μm | Higher frequencies |
Micro-CT | 1–2 mm | ≤200 μm | Higher X-ray beam, smaller focal spot |
MRI | ~1 mm | ≤100 μm | Higher field intensity, improved gradient fields and coils |
SPECT | ~1 cm | 0.5–2 mm | Pinhole collimation |
PET | ~5 mm | 1–2 mm | Reduced detector-element size, smaller-diameter detector rings |
In addition, the mouse heart beats are about 400–600 beats per minute, and respiration frequency ranges from 30 to 60 per minute. In vivo imaging has to take into account cardiac and respiratory motion artifacts present during acquisition. A temporal resolution of about 50 ms or a monitoring system is necessary for motion-compensated imaging.
High-resolution in vivo imaging studies are not intended to supplant histological analysis but allow investigating small animal’s anatomy, pathology, and development offering two significant advantages: (1) the acquisition of data noninvasively and longitudinally from the same live animal and (2) the regulation of the number of animals used in studies as suggested by the 3 Rs principle (reduction, replacement, and refinement). For these reasons, the imaging protocol must have negligible effects on the animal health. In vivo imaging requires the animal to be restrained during acquisition using anesthesia system [30]. The anesthesia as well as radiation dose or contrast agent injection must be carefully selected [31].
The researcher has to choose which imaging modality is suitable to his mouse model and problematic. These various imaging techniques are complementary and assess different parameters as organ morphology (size, volume, localization, lesion size, or evolution), organ functions (physiology, metabolism, etc.), cellular functions, or molecular processes (Fig. 1) [11]. In some cases, multimodality approaches may be employed. Nevertheless, these techniques are different in terms of acquisition time, sensitivity, spatial resolution, ease of use, and cost (Fig. 2).



Fig. 1
Complementarity of the different imaging modalities

Fig. 2
In vivo imaging modalities in mice: a sensitivity, resolution, invasivity, and cost comparison. Sensitivity refers to the in vivo concentration of the labeled contrast agent (probe) required to obtain a certain signal-to-noise ratio. US refers to high-frequency ultrasound imaging; aMRI, fMRI, and MRS to anatomical, functional magnetic resonance imaging and magnetic resonance spectroscopy; CT to computed tomography; SPECT and PET to single photon emission computed tomography and positron emission tomography; and FLI and BLI to fluorescence and bioluminescence imaging
All imaging modalities are based on the use of electromagnetic radiations, with the exception of ultrasound imaging using acoustic waves.
Electromagnetic radiations are energy transported through space in the form of periodic sinusoidal waves containing both electrical and magnetic fields and can be described in both time and space. An electromagnetic wave propagates at the speed of light and is characterized by its frequency expressed in inverse seconds (/s) or hertz (Hz) or its wavelength expressed in nanometers (10−9 m). These two quantities are related to the speed of light by the equation:


The frequency (and hence, the wavelength) of an electromagnetic wave depends on its source. There is a wide range of frequencies encountered in our physical world; this constitutes the electromagnetic spectrum (Fig. 3).


Fig. 3
The electromagnetic spectrum. It can be divided into frequency and hence wavelength regions, ranging from the low frequency of the electric waves generated by the power transmission lines to the very high frequency of the gamma rays originating from the atomic nuclei. Each imaging modality uses portions of the electromagnetic spectrum
Sound waves propagate at a speed depending on the medium they cross. The speed of sound is equal to the product of wavelength and frequency.
All imaging modalities have the same processing: (1) Electromagnetic radiations cross the body and interact with the biologic matter, (2) the output radiations after these interactions are detected with particular instrumentations and produce analog signals, and (3) these signals are digitalized and processed to create an image. The two-dimensional (2D) image is composed of pixels (picture elements) or voxels (volume elements) in three-dimensional (3D) representation including the slice thickness (Fig. 4).


Fig. 4
Medical imaging process
In vivo image is not a simple “picture” of the studied tissues but a visual representation of some of their specific chemical and physical characteristics. The following chapters will give an overview of the basic principles of each in vivo imaging modality and will provide some applications on mice.
2 High-Resolution Ultrasound Imaging
Principle: High-resolution ultrasound (US) imaging or ultrasound biomicroscopy (UBM) is a noninvasive technique based on the use of high-frequency sound waves not detectable by human ears (higher than 20 kHz) to produce dynamic images of organs, tissues, or blood flow (Doppler technique) inside the body.
2.1 Generation of an US Wave
US wave is generated when an electrical current is applied to an array of piezoelectric crystals located on the transducer surface. Electrical stimulation causes mechanical distortion of the crystals (expansion and contraction) resulting in vibration and production of sound waves. The conversion of electrical to mechanical sound energy is called the piezoelectric effect. Each piezoelectric crystal produces an US wave, and their summation forms the US beam. US waves are generated in pulses (intermittent trains of pressure waves).
2.2 US Tissue Interaction
The propagation speed of sound varies in different biological media and is dependent on the acoustic impedance of the medium which is the resistance of a tissue to the passage of US. In most soft tissue, the speed average value is supposed to be constant at 1,540 m/s, and this is the value assumed by US machines for all biologic tissues [32, 33].
When the US beam crosses tissues, the amplitude of the original signal is attenuated as the depth of penetration increases. This loss of energy or attenuation is due to absorption in homogeneous media or reflection, refraction, and diffraction at tissue interfaces, e.g., between two tissues with different acoustic impedances (Fig. 5).


Fig. 5
US tissue interaction (a) in an homogeneous medium, (b–d) at tissue interface
Absorption of US wave into a homogeneous medium corresponds to the conversion of acoustic energy to heat. The absorption coefficient is proportional to the square of the frequency, then to explore deep regions as abdomen, only low frequencies (3.5 MHz) are used.
Transmission and reflection occur on interfaces. When an incident US wave hit an interface, a part of US energy depending on the degree of impedance mismatch between the two tissues is reflected. The reflected US waves, called echoes, can be detected by the transducer and forms the basis of ultrasound imaging.
Refraction occurs when an incident wave hits the interface at an angle inferior to 90°; the penetrating pulse direction will be shifted by the refraction process. The transmitted wave propagates in a medium where the speed is not the same; the transmitted angle is different to the angle of incidence (Snell’s law).
Diffusion occurs when the incident wave encounters an interface that is not perfectly smooth (e.g., surface of visceral organs) or when the wavelength of the US wave is larger than the dimensions of the reflective structure (e.g., red blood cells). The reflected echoes scatter in many different directions resulting in signals of similar weak amplitudes.
2.3 Formation of an US Image
The US pulses emitted from the transducer are transmitted into the body, reflected on tissue interfaces, and returned to the transducer (echoes). They are then converted into an electric signal which is processed and displayed as an image on the screen using a gray scale.
The degree of brightness, e.g., echogenicity, represents the amplitude of echoes which returns to the transducer (Fig. 6). There is a large difference between the acoustic impedance of bone and any soft tissues, leading to a hyperechoic image. Air and any soft tissue interface or steel interface also give a hyperechoic image. It is clinically important to apply a conducting gel (an acoustic coupling medium) on the transducer surface to minimize this difference.


Fig. 6
Tissue appearance under US
2.4 Instrumentation
A typical ultrasound machine consists of a transducer (probe used to send and receive the US waves by converting electrical energy to mechanical (ultrasound) energy and vice versa) and a pulse control unit to change the amplitude, the frequency, and the duration of the emitted pulses. The micro-ultrasound systems operate at frequencies of 30–100 MHz compared to frequencies of 2.5–15 MHz for clinical systems to improve spatial resolution.
2.5 Different Types of Ultrasounds
Conventional US machine displays 2D images or slices of the body (2D B-mode—brightness mode) and 3D reconstruction. Four-dimensional (4D) ultrasound produces a moving picture version of 3D ultrasound (M-mode—motion mode). A Doppler ultrasound study (power Doppler mode to detect and map the microcirculation or color-flow Doppler mode to visualize the speed and direction of blood flow through the blood vessels [34–36]) may be part of an ultrasound examination.
The Doppler effect occurs when there is a moving source (blood flow of red blood cells) and a stationary receiver (US transducer). The relative motion between the sound source and the receiver changes echo frequencies, e.g., if the source is moving toward or away from the transducer, the perceived frequency is higher or lower than the actual one.
2.6 Applications
UBM is a noninvasive real-time technique that provides accurate and reliable images in embryos to adult mice of almost the organs observed in humans.
Contrast agents as air-filled microbubbles can be injected intravascularly as a bolus into tissue. These microbubbles have a highly hyperechoic appearance resulting in an increased contrast due to the high echogenicity difference between air and soft tissues. This technique is called contrast-enhanced US imaging (for review, see [37]).
2.6.1 Developmental Biology
UBM is the unique modality able to phenotype live mouse embryos in utero. Since its first report in 1995 by Turnbull and collaborators [38], UBM has been applied in various applications, mostly for brain and cardiovascular imaging (reviewed in [36, 39]). UBM images provide phenotype analysis of mutant and transgenic mice from embryonic to adult stages and have sufficient resolution to visualize small morphological structures at early embryonic period E11.5 [40] and numerous organs under development such as amniotic membrane and cavity, yolk sac, placenta, umbilical vessels, and heart and brain ventricles [36].
Some investigations have focused on the examination of mouse cardiovascular development [41–43] and on the use of UBM-guided injections of cells and retroviruses to specific locations in developing mouse embryos [39, 44–48] (for review, see [49]). An example of US-guided microinjection into the mouse forebrain in utero at E9.5 is shown in Fig. 7.


Fig. 7
Viral injection into the E9.5 mouse forebrain ventricles. (a) The anesthetized animal is placed on the injection stage. Two embryos would be exposed and positioned under the ultrasound probe where indicated (arrow). The microinjection needle filled with virus is located near the embryos and lined up with the ultrasound probe. (b) Screen capture of a real-time US image from an E9.5 mouse embryo. V ventricle, AS amniotic sac, UW uterine wall. (c) Embryos were injected with a PLAP-expressing virus at E10.5. Histochemical staining for PLAP reveals the location of viral infection events. Reproduced from [Pierfelice and Gaiano 2010] with permission from JOVE [48]
UBM in Doppler mode has enabled analysis of blood flow properties in the embryo [42, 46] and cardiovascular function evaluation (for review, see [40, 50]). Recently, UBM in color-flow mode, in which Doppler signals are analyzed in real time, allows producing a color-coded map of blood velocities [49, 51].
2.6.2 Oncology
UBM is strongly used to study mouse cancer models particularly in xenograft models allowing the accurate assessment of tumor size at early stage and noninvasive longitudinal investigations on tumorous development, growth, and differentiation and on therapy effects [52, 53] (for review, see [36]). Recently, UBM has been useful to detect apoptotic cell death in a model of melanoma [54].
Tumor vascularization can be visualized on Doppler mode with good sensitivity for vessels larger than capillaries to assess neoangiogenesis [32, 36, 55]. The first 3D power Doppler ultrasound imaging was recently reported by Xuan et al. in a genetically engineered mouse prostate cancer model [56]. Contrast-enhanced micro-CT with microbubbles improves Doppler signal intensity.
2.6.3 Cardiology
Echocardiography is an important tool to noninvasively assess the phenotype of genetically and surgically altered mice and allows anatomical and physiological characterization of the heart and great vessels.
UBM has been used to visualize cardiac structure, valve morphology, and function analysis in mouse embryos [42] and in adult mice (for review, see [61]); to calculate cardiac function parameters such as stroke volume, cardiac output, ejection fraction, and fractional shortening (reviewed in [62]); and to evaluate left ventricular (LV) structure and systolic and diastolic function and calculate LV mass [63, 64] in normal or in models of cardiovascular diseases and in pharmacological treatments [33, 36].
High-resolution echocardiography is able to guide catheter injections or cardiac injections of labeled cells accurately into the mouse myocardial wall [65, 66] (for review, see [61]).
Microbubble contrast agents are currently used in echocardiography to enhance the echogenicity of the cardiac chamber and in myocardial perfusion assessment [36].
2.6.4 Ocular and Dermal Applications
UBM is able to evaluate anterior ocular segment anatomy and its pathology such as corneal disease and trauma, glaucoma, cysts, and tumors, to perform some quantitative analysis, and to assess posterior structures of the eye such as the retina or optical nerve (for review, see [32, 36]).
3 Micro X-Ray Computed Tomography
Principle: X-rays crossing the tissues are attenuated at different rates depending on the tissue density and then picked up by detectors placed on the opposite end of the CT scanner from the emission X-ray source [67–69].
3.1 X-Ray Interaction with Matter
X-ray CT is made possible by the relative transparency of X-rays through the body. As an X-ray beam crosses the biologic matter, it can (1) transmit through the matter without any interaction (primary or direct radiation), (2) interact with the matter and be completely absorbed by depositing its energy (photoelectric effect), and (3) interact and be scattered or deflected in a new direction and deposit a part of its energy (secondary radiation, Compton effect) (Fig. 8).


Fig. 8
Principal physical processes in X-ray attenuation in the matter: In red, no interaction. In green, photoelectric absorption: the incident X-ray beam is totally removed with transfer to the matter all of its energy. In blue, Compton scattering interactions: the X-ray beam is diverted in a new direction, with or without loss of energy transferring to the matter
The total attenuation is the sum of the attenuation due to different types of X-ray interactions with the matter. It depends on the sample thickness, its density, its atomic number, and the X-ray energy (Beer’s law).
3.2 Generation of an X-Ray Image
An X-ray image or projection represents an image of the sum of all local attenuations of an X-ray beam crossing the biological sample. To create a 2D or 3D tomographic image, a set of measured projection data need to be acquired. These projection views are acquired at different equally spaced angular positions around the rodent. A filtered back-projection algorithm [67, 70] is then used to reconstruct the distribution of X-ray attenuation in the slice plane. The reconstructed image is a spatial map of measured attenuation coefficients, e.g., each pixel in the reconstructed image is expressed as CT numbers in Hounsfield unit (HU) [67]. CT numbers are relative to water attenuation, which is assigned to a value of 0 HU. The highest CT number appears white on the CT image (e.g., in bones), and the lowest CT is black (for air); between these extremes are various shades of gray (Fig. 9).


Fig. 9
A representation of Hounsfield’s scale. Air is assigned a value of −1,000 HU and bone a value of +1,000 HU; water is the reference at 0 HU. The gray scale color depends on the selected window setting; the bottom scale shows the gray scale at level 0 and window width of 200. Thus, the highest number is usually assigned to white and the lowest number to black
3.3 Instrumentation
The basic components of a micro-CT scanner are a micro-focus X-ray tube with focal spot diameter allowing high isotropic spatial resolution down to a range of 10–50 μm [68, 71] and a high-resolution X-ray detector, consisting of a charge-coupled device (CCD) array optically coupled to a phosphor screen via an optical lens or an optical fiber [68, 72, 73] or an X-ray image intensifier (XRII) screen coupled to a video readout [17].
The different projections are acquired by rotating synchronously the X-ray source and the detectors array around the animal using either a rotational bed device and stationary X-ray source and detectors (mainly used for in vitro imaging) or a rotational gantry and stationary sample design [21, 68, 71, 74].
3.4 X-Ray Dose
3.5 Applications
Micro-CT can be used for noninvasive imaging of various organs such as skeleton, chest, abdominal organs, and brain [21].
3.5.1 Bone Imaging and Phenotype Evaluation
Bones provide a very strong contrast, e.g., the attenuation difference between bone and soft tissue is large so that micro-CT is the predominant technique used to study bone microarchitecture and density. 3D reconstruction led to measurements of trabecular bone morphology descriptors such as bone volume ratio, trabecular thickness, spacing, density, or connectivity in pathology such as osteoarthritis, bone metabolism, and gene-engineered mice skeleton [16, 21, 68, 69, 74, 79]. 3D micro-CT can be used to image and display the whole macroscopic mouse skeleton to study skeletal development and to screen bone metastases [68, 69, 80, 81].
Skeletal structure investigations in transgenic and in knockout mice assess phenotypic parameters of skeletal or soft tissues such as muscle thickness or fat [74].
3.5.2 Lung and Heart Imaging
Lung or heart visualization is a challenge in living mouse thoracic micro-CT imaging because of its small size and rapid movements generating motion artifacts [21, 68, 69]. Nevertheless, the large contrast at air/soft tissue interfaces makes micro-CT a method of choice for lung imaging.
With improved technologies such as respiratory gating (widely described by Badea and coworkers [82–86 and [87–89]) or iso-pressure breath hold [90, 91], it is possible to investigate pulmonary anatomy and function in normal, acute, or chronic disease mice such as emphysema and fibrosis [85, 87, 89, 92–96].
The thoracic structure including heart, esophagus, trachea, bronchi, and large vessels can be imaged [68].
Longitudinal studies of lung tumor size or tumor angiogenesis can be performed [68, 69, 89, 92, 97–99]. An example of angiogenesis in a xenograft mouse model of lung cancer is illustrated in Fig. 10.


Fig. 10
3D surface rendering images of lung tumors from untreated and bevacizumab-treated mice. Extracted tumors and tumor microvascularization are shown (yellow represents tumor tissue area; red represents tumor vessel area). Reproduced from [Savai et al. 2009] with permission from Neoplasia [99]
3.5.3 Vascular Imaging
Blood vessels provide a little contrast so that vascular contrast agents must be employed. Most X-ray contrast agents are based on the nonionic, water-soluble, extracellular element iodine and are intravenous injected. The microvascular anatomy can be visualized in both in vivo and ex vivo studies with a spatial resolution less than 50 μm using blood-pool contrast media; discussed in [68, 69].
Micro-CT allows 3D views of the entire microvascular structure, and 3D connectivity might be quantitatively investigated.
Recently, Chugh and collaborators describe the application of micro-CT to measure the cerebral blood volume for characterizing total vascularity in 3D regions of the mouse brain [101].
3.5.4 Soft Tissue Imaging
As described in physical basis section, the soft tissue contrast is relatively weak, but fat differentiation is possible. Fat volume measurements are of great interest in mouse disease models such as diabetes [68, 70]. Muscle volume becomes also feasible and can be useful in musculoskeletal disorder characterization.
Organs in the peritoneal cavity such as liver, kidney, spleen, heart, lung, stomach, adrenal gland, gut, and bladder can be identified, and their boundaries or peritoneal tumors can be accurately delineated using iodinated contrast agent injected into the peritoneal cavity [68].
4 Magnetic Resonance Microscopy
Principle: Magnetic resonance microscopy (MRM) involves the nuclear magnetic alignments of different atoms inside a magnetic field to generate images.
4.1 Basics of MRI: Physics and Instrumentation
4.1.1 Nuclear Magnetic Resonance (NMR)
As other nuclei, the nucleus of hydrogen atom has magnetic properties called spin: it behaves like a little magnet spinning around itself and can be represented as a vector. As all organic tissues contain about 70 % of water, the hydrogen nucleus is very abundant and is mainly used in magnetic resonance imaging (MRI).
In a static magnetic field Bo, spins rotate around Bo direction with a frequency fo (in radiofrequency (RF) range). This rotation, named Larmor precession, is described in the following formula: ωo = 2πfo = γBo, where γ is the gyromagnetic ratio, depending on the nucleus type. A magnetization vector (sum of all spin vectors in the body) is so created along the longitudinal axis (Bo or Z axis).
An RF field is then applied for a short time (RF pulse) via a transmit coil to the magnetization which is tipped away from the Bo direction to the transverse plane (perpendicular to Bo axis). This RF pulse is tuned to the Larmor frequency to deliver the maximum energy to the spins (resonance phenomenon).
At the end of the RF pulse, the magnetization tends to realign to the Bo direction, describing a spiral trajectory called relaxation. The return of the magnetization along Bo direction induces a small electric signal in a receive coil near the body, the free induction decay (FID).
They are two main features of the relaxation phenomenon: a dephasing of the spins, perpendicularly to Bo direction, named T2 or transverse relaxation, and a realignment along the Bo direction, named T1 or longitudinal relaxation. Both of these relaxations produce a signal which leads to different contrast images [102, 103].
4.1.2 From Signal to MR Image
To get an image, a spatial encoding is accomplished by superimposing three linear field gradients (in the X, Y, and Z directions) to the permanent field Bo. According to the Larmor precession formula, gradients slightly modify linearly the magnetic field intensity resulting in a change in resonance frequency. The MR signal is then coded in a so-called k-space or spatial frequency space with an appropriate frequency and phase for each point. A mathematical operation (Fourier transform) allows obtaining an image from this k-space [102, 104].
The RF pulses, their duration, the time between them, and the gradients application are part of a program called a sequence.
4.1.3 Contrasts on MR Images
The major contrasts obtained on MR images are proton density and T1 and T2 weighting. Signal intensity is then expressed for each weighting in gray scale (Fig. 11). MRI uses also the relaxivity properties of contrast agents like gadolinium to highlight specific tissues [105].


Fig. 11
Tissue appearance on MR images
4.1.4 Instrumentation
A conventional MR spectrometer uses a supraconductive high-field magnet (Bo intensity up to 4.7 teslas for small animals), emission and/or reception coils (conductive wire with different shapes according to the organ to scan and its dimensions) to receive and/or transmit the RF signal, and powerful gradient coils to provide spatial localization of the signal [102].
4.2 Overview of MR Techniques
4.2.1 Anatomy
Anatomical MRI is based on proton density-, T1-, or T2-weighted images which have a good contrast in soft tissues. It is useful to visualize anatomical structure of organs, to make volumetric quantification, and to see subtle details in healthy animals or in genetically altered models (Fig. 12) [106–110].


Fig. 12
Anatomical resolution in C57BL6/J mouse brain images acquired in vivo and in vitro with different MR contrast techniques. Left: in vivo T2-weighted RARE (rapid acquisition with relaxation enhancement) sequence, 100 μm × 100 μm × 100 μm, 9 × 4 T. Middle: in vivo diffusion-weighted spin-echo image, 0 × 009 mm3, 9 × 4 T. Right: in vitro T2*-weighted image, TR (repetition time) = 150 ms, TE (echo time) = 7 × 5 ms, 0 × 001 mm3, 17 × 6 T. Reproduced from [Benveniste 2006] with permission [108]
4.2.2 Functional Imaging
Functional MRI (fMRI) is mainly based on vascular variations due to neuronal activity. The T2*-weighted image intensity is influenced by the oxygenation state of the blood (blood oxygenation level-dependent (BOLD) effect) [111].
4.2.3 Diffusion and Diffusion-Tensor Imaging (DTI)
Diffusion MRI (dMRI) allows the noninvasively mapping of the diffusion process of water molecules in biological tissue. Molecular diffusion in tissues is not free, reflecting interactions of molecules with many obstacles, such as macromolecules, fibers, membranes, etc. Water molecules are thus used to reveal microscopic details on tissue architecture, either in a normal or diseased state.
4.2.4 MR Angiography
Blood flow causes disruption of spatial encoding and artifacts. The sensitivity of MRI has been used to develop vascular imaging techniques using the physical changes associated with flow: time of flight and phase contrast. Contrast agents are often used in MR angiography.
4.2.5 Perfusion
In MRI, perfusion provides access to information on the microcirculation in capillary tissue. The main quantitative parameters estimated by perfusion imaging are blood volumes, cerebral blood flow (CBF), and temporal data (transit time, time to peak contrast, etc.).
4.2.6 MR Spectroscopy
MR spectroscopy (MRS) allows noninvasive in vivo analysis of the molecular composition of tissues. It identifies certain molecular components, metabolites, involved in physiological or pathological processes. Proton MRS uses the signal from hydrogen nucleus to determine the concentration of brain metabolites such as N-acetyl aspartate (NAA), choline (Cho), creatine (Cr), and lactate (Lac) in the tissue examined. MRS can also be achieved with other nuclei including 31P, 13C, 15N, 23Na, and 19F [112, 113].
4.3 Applications
4.3.1 Brain Imaging
Imaging the rodent brain at high resolution is particularly useful in studies of genetically manipulated mice and provides valuable morphologic information about neurological diseases that are often characterized by subtle structural changes [114]. A continuing challenge in brain imaging, however, is to create sufficient contrast, because conventional contrast agents do not penetrate the blood–brain barrier (BBB). Highly specialized techniques and often exogenous contrast agents are necessary to reveal structural detail based on nuclei, fiber tracts, and ventricular spaces. Nevertheless, MRM is well suited to anatomical phenotyping of genetically manipulated organisms such as transgenic mice as models of human neurological diseases [115, 116]. Proton MRS also provides a noninvasive way to investigate in vivo neurochemical abnormalities. An example in transgenic mouse models of Alzheimer’s disease is displayed in Fig. 13.


Fig. 13
Representative image and spectra of mouse brain. (a) An image of mouse brain with placement and size of the voxel. (b–e) Localized in vivo 1H MR spectra obtained from 18-μl voxel (box in a) from the brain of 23-month-old B6/SJL wild-type (WT) mouse b and an APP-PS1 mouse scanned at 16 c, 20 d, and 23 e months of age. The spectra are shown with similar linewidths and with amplitude adjusted by using the total creatine (tCr) peak at 3.03 ppm. Reproduced from [Marjanska 2005] with permission [112]
Construction of MRM atlases of the brain for inbred mouse strains in the developing embryo and adult is in progress [109, 117, 118] [http://mouseatlas.caltech.edu/].
At high field, fMRI on a mouse somatosensory stimulation model could be routinely performed and could be applied on transgenic mice [119].
4.3.2 Oncology
DWI in rodents has been applied using models in tumor research. The apparent diffusion coefficient (ADC) value has been found to negatively correlate with tumor cellularity. MRS studies are performed on large tumors, for example, on hepatocellular carcinoma [120] or prostate cancer [121, 122].
MR angiography can be used to observe malignancy-associated vessel tortuosity using a model of choroid plexus carcinoma in genetically engineered mice [123].
Perfusion studies analyzing the capillary compartment of the brain, the liver, and the kidneys have been carried out successfully using clinical scanners. Tumoral vessel density and perfusion are parameters of great interest, as they are considered to be surrogate parameters for angiogenesis.
4.3.3 Cardiovascular Imaging
Cardiovascular research relies on small animals like mice which allow study of phenotypic expressions of genomic alterations and exploration of different therapeutic approaches. The involvements of the arterial, venous, and capillary system are numerous particularly in diseases like atherosclerosis, thrombosis, angiogenesis, diabetes mellitus, moyamoya, and aneurysms. MRI imaging has been done on both magnetically labeled mouse embryonic stem cells and their restorative effects on cardiac function in a murine model of acute myocardial infarction [124].
5 Nuclear Imaging
Principle: A radioactive tracer is injected and then fixed to a specific organ or molecule; its radiations are detected and converted to an electrical pulse. Nuclear imaging can be used to study the activity of cell metabolism.
5.1 Radioactivity Properties
Nuclei, composed of protons and neutrons, can become unstable when the number of neutrons is lower than the number of protons or when these numbers are too important. An unstable nucleus will lose its excess energy by the emission of particles. This phenomenon is called nuclear radiation.
There are three types of nuclear radiations:
α radiation corresponds to the emission of helium nuclei. This radiation is too dangerous to be used for nuclear imaging.
β radiations correspond to the emission of either an electron and a neutrino (for β− radiation) or a positron and an antineutrino (for β+ radiation). β+ radiation, used in PET imaging, induces a positron which is like an electron with a positive charge. This kind of antimatter particle is very unstable and tends in an ultra short time to be annihilated by the first electron it meets, creating two γ photons emitted in opposite directions and with the same energy of 511 keV.
γ radiation, used in SPECT imaging, corresponds to the emission of energized photons due to the rearrangement of the nuclei particles. γ radiation generally follows α or β radiations.
The unstable nuclei used in nuclear imaging are obtained artificially with a particle accelerator, called a cyclotron, by hitting a target with accelerated charged elementary particles. These radioisotopes are then chemically fixed to a biological vector and can be injected in tissues to be imaged.
5.2 SPECT (Single Photon Emission Computed Tomography) or Scintigraphy
SPECT or scintigraphy consists in collimating with specialized submillimeter pinholes and detecting the γ radiations emitted by radioisotopes after their injection and fixation to the interest organs. For this purpose, γ detectors scan the body and change the number of γ photons detected to an electrical signal.
A γ detector (Fig. 14) is made up of a scintillator which transforms a γ photon into a visible photon and a photomultiplier which transforms a visible photon into an electron and multiply then the number of electrons. The scintillator is composed of a crystal; the detector sensitivity and the imaging system spatial resolution will depend on the type and the size of this crystal. Several research groups have developed high-resolution detectors using new materials like plastics or solid-state detectors [125, 126]. The photomultiplier is first formed by a layer alkaline which has the property of changing a visible photon into an electron. This electron enters into a vacuum chamber and is attracted by a first anode covered with alkali oxide, which produces several secondary electrons. These electrons are again attracted by a second anode and multiplied and so on until an electrical signal can be detected.


Fig. 14
γ detector principle
Dozens of γ detectors are associated in a network of resistors or capacitors to form the γ camera used in scintigraphy. This γ camera can be static and allows obtaining images of radioisotope concentration in an organ with a submillimeter resolution. It is then possible to acquire successive static images with a minimal temporal resolution of about 1 s to study radioisotope bio-distribution (the image intensity is proportional to the tracer concentration) and then quantify the molecular kinetic processes in which they participate [127]. The γ camera can also move along the body to obtain a larger image or a tomography. The tomography consists in the acquisition of a succession of static images with different angle orientations. The γ camera turns around the body with the imaged organ placed at the center of the rotation to keep the same distance between the γ photon source and the receptors. A matrix computation (analogue to the scanner X) provides axial, sagittal, and coronal slices as well as three-dimension volumes.
5.3 PET (Positron Emission Tomography)
A PET scanner consists of one or more γ detector crowns placed around the body. When a β+ radioisotope is injected, the two γ photons resulting from the interaction of the positron emitted with the nearest electron are detected in coincidence by two γ detectors facing.
Before the radioisotope injection, an attenuation map is acquired using a γ source moving around the body. On the PET–CT systems, the γ source is replaced by the X-ray source. This map allows correcting the effects of attenuation or diffusion (when a γ photon is respectively stopped or deflected by tissues before achieving a γ detector).
Then, the signal from all the detectors is registered into a big matrix called a sinogram and processed. Firstly, the photons whose energy is different from 511 keV are removed. Secondly, the coincidence detection allows only virtually simultaneous detection (less than 15 ns difference) of two γ photons by two detectors facing. This determines the line of response (LOR) where the emission source should be located. Thirdly, the position of the source on the LOR is calculated by measuring the delay between the two detections (in fraction of nanosecond); this is the time of flight technique. Electronic and computer parts of a micro-PET scanner are adapted to these high-precision timing measurements (Fig. 15). The signal is also corrected taking into account the sensibility and the efficacy of each detector. Images of multiple slices or 3D volumes are then calculated using a mathematical transformation. Scanners now achieve 1 mm resolution and more than 3 % sensitivity [10].
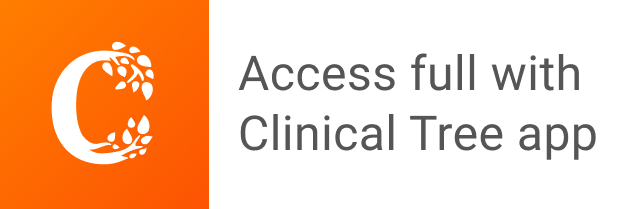