Fig. 20.1
Mechanism of generation of reactive oxygen species and oxidative stress. Leaked electrons from mitochondrial ETC complexes I and III react with molecular oxygen to produce superoxide (first free radical). The superoxide is then converted to hydrogen peroxide (H2O2) by superoxide dismutase (SOD). Superoxide can also react with nitric oxide (NO) to form peroxynitrite (ONOO•), and it can also contribute to the formation of hydroxyl (OH•) radical through Haber-Weiss and Fenton reactions. On the other hand, antioxidant enzymes (catalase and glutathione peroxidase, GPx) can convert H2O2 to H2O. Glutathione (GSH) is a major antioxidant, which is converted to its oxidized form (GSSG) by GPx. A highly potent free radical, OH• oxidizes lipids, proteins and nucleic acids. Myeloperoxidase (MPO) can also convert H2O2 in the presence of chloride to hypochlorous acid (HOCl), which can react with Fe2+ and superoxide to form OH•. HOCl can also get converted to singlet oxygen.
Mitochondrial ETC complexes I and III are the main sites of production of superoxide radical by mitochondria (Barja 1999; Muller et al. 2004). The leaked electron reduces molecular oxygen to superoxide anion, which is an initial step for subsequent production of other ROS. Superoxide anion can be converted to H2O2 by superoxide dismutase (SOD). Alternatively, H2O2 can also be produced by the actions of xanthine oxidase (XO), MAO or nicotinamide adenine dinucleotide phosphate (NADPH)-oxidase (Granger 1988; Dupuy et al. 1991). It can get further converted to highly reactive hydroxyl radicals, which requires a two step reaction (Fig. 20.1) . In the first step (I), superoxide gives rise to molecular oxygen by Haber-Weiss reaction where Fe3+ is converted to Fe2+. In the second step (II), Fe2+ converts H2O2 to hydroxyl radical by Fenton reaction. In the reaction catalyzed by myeloperoxidase (MPO), H2O2 can also react with chlorides to form hydrochlorous acid (HOCl), which can either get converted to singlet oxygen, or to hydroxyl radicals by reacting with Fe2+ or superoxide. In addition, superoxide can also react with nitric oxide (NO) resulting in the formation of peroxynitrite (ONOO·) .
There is substantial evidence suggesting that environmental factors can increase vulnerability to oxidative stress, and may lead to clinical phenotype in autism. Our previous reviews have discussed the role of endogenous and exogenous environmental pro-oxidants such as NO, XO, homocysteine, heavy metals (mercury, lead), maternal prescription drugs (thalidomide and valproic acid), air pollutants, chemicals and toxins, pathologenic bacteria and viral infections that can lead to oxidative stress in autism (Chauhan and Chauhan 2006; Chauhan et al. 2009a) .
It is widely accepted that an excess of ROS is toxic and damages essential cell components such as nucleic acids, proteins and lipids, leading to cell death. ROS can lead to oxidation of amino acid side chains, formation of protein-protein cross-linkages, and oxidation of the protein backbone resulting in protein fragmentation. Although all DNA bases are susceptible to damage, guanine is most prone to ROS-mediated oxidation of DNA and is therefore a primary target of oxidative modification (Cadet et al. 2002). Brain is a rich source of polyunsaturated fatty acids, which are easy targets of oxidation . The most common oxidative markers of protein oxidation are protein carbonyls and protein nitrates. The common markers of lipid peroxidation by free radicals are malonyldialdehyde (MDA), 4-hydroxynonenal (HNE), and F2-isoprostanes. The nucleic acid oxidation products that are frequently used for measurement of oxidative stress are: 8-hydroxy deoxyguanosine for DNA, and 8-hydroxyguanosine for RNA .
20.2 Antioxidant Defense
Antioxidants can be enzymatic or non-enzymatic in nature As shown in Fig. 20.1, major enzymes that participate in anti-oxidant activity include SOD, catalase and glutathione peroxidase (GPx) . Non-enzymatic antioxidants include redox proteins such as thioredoxins, peroxiredoxins, and glutaredoxins (Birben et al. 2012), and low molecular weight compounds such as glutathione (GSH), vitamin C, vitamin E and beta-carotene. Among these antioxidants, GSH is a major endogenous antioxidant that protects cells from endogenous and exogenous toxins, particularly in the central nervous system.
20.3 Oxidative Stress in Autism
Oxidative stress in autism can be caused by increased ROS generation, decreased anti-oxidant defense (enzymatic or non-enzymatic), and mitochondrial dysfunction .
20.3.1 Increased ROS-Mediated Oxidative Damage in Autism
It has been suggested that increased vulnerability to oxidative stress by endogenous or environmental pro-oxidants in conjunction with genetic susceptibility factors may contribute to the development and clinical manifestations of autism (Chauhan and Chauhan 2006; Kern and Jones 2006; Deth et al. 2008; Chauhan et al. 2009a) . In support of this hypothesis, there are numerous reports indicating increase in markers of lipid peroxidation, protein oxidation, and DNA oxidation, and reduced antioxidant status in blood and urine samples and in the post-mortem brain tissue samples from subjects with autism compared with age-matched control subjects .
We reported increased levels of MDA, a marker of lipid peroxidation, in the plasma of children with autism compared to their typically developing siblings (Chauhan et al. 2004), and also increased lipid peroxidation (Muthaiyah et al. 2009; Chauhan et al. 2011b), DNA oxidation (Chauhan et al. 2011a), and protein oxidation (Chauhan et al. 2010) in the cerebellum and frontal and temporal regions of the brain in the subjects with autism as compared to age-matched control subjects. Oxidative stress in autism is brain-region specific because increased lipid peroxidation, DNA and protein oxidation were not observed in parietal and occipital cortices of autistic subjects compared with age-matched control subjects (Muthaiyah et al. 2009; Chauhan et al. 2011b; Chauhan et al. 2011a; Chauhan et al. 2010). Other studies have also indicated increased levels of lipid peroxidation and protein oxidation markers in autism, i.e., increased thiobarbituric acid (TBA)–reactive substances in the erythrocytes of autism subjects (Zoroglu et al. 2004) ; increased excretion of F2-isoprostane, and 8-OH deoxyguanosine in the urine of children with autism (Ming et al. 2005); increased levels of lipid-derived oxidative protein modification, i.e., carboxyethyl pyrrole and iso 4-levuglandin E2-protein adducts, in the brain, primarily in the white matter of autistic subjects (Evans et al. 2008); increased levels of 3-nitrotyrosine (a specific marker for oxidative damage of protein) and 8-OH deoxyguanosine in the cerebellum and temporal cortex of autistic subjects (Sajdel-Sulkowska et al. 2009; Rose et al. 2012a) . The density of lipofuscin, a matrix of oxidized lipid and cross-linked protein, was also observed to be greater in the cortical brain areas involved in social behavior and communication in autism (Lopez-Hurtado and Prieto 2008). Recently, Pecorelli et al. (2013) reported increased levels of HNE in the erythrocytes and plasma of children with autism as compared to controls .
20.3.2 Glutathione Redox Imbalance in Autism
Glutathione is the most important endogenous antioxidant for detoxification and elimination of environmental toxins and free radicals . Several studies have reported lower levels of reduced glutathione (GSH), higher levels of oxidized glutathione (GSSG), and a lower redox ratio of GSH/GSSG in the plasma of individuals with autism (James et al. 2004, 2006; Al-Gadani et al. 2009; Geier et al. 2009; Bertoglio et al. 2010; Adams et al. 2011a, b). Al-Yafee et al. (2011) also reported reduced levels of GSH, GSH/GSSG, and increased levels of thioredoxins and thioredoxine reductase in the plasma of children with autism as compared to controls. In a systemic review and metanalysis of oxidative stress-related markers in autism, Frustaci et al. (2012) reported that ASD subjects had reduced levels of GSH, GPx, methionine and cysteine by 27, 18, 13 and 14 % respectively, and increased concentration of GSSG by 45 % in the blood. Pecorelli et al. (2013) observed decreased levels of GSH in the erythrocytes in the subjects with autism as compared to control subjects. Other studies have also demonstrated decreased ratio of GSH/GSSG in the lymphoblastoid and primary immune cells from autistic subjects (James et al. 2009; Rose et al. 2012b) .
We have reported brain-region specific glutathione redox imbalance in autism (Chauhan et al. 2012a). Reduced levels of GSH, increased levels of GSSG, and a decrease in the ratio of GSH/GSSG were observed in the cerebellum and temporal cortex of autistic subjects compared with age-matched control subjects (Chauhan et al. 2012a). GSH levels were significantly decreased by 34.2 and 44.6 %, with a concomitant increase in the levels of GSSG by 38.2 and 45.5 %, respectively in these brain tissues in autism, as compared to the control group . There was also a significant decrease in the levels of total GSH (tGSH) by 32.9 % in the cerebellum, and by 43.1 % in the temporal cortex of subjects with autism. The redox ratio of GSH to GSSG was significantly decreased by 52.8 % in the cerebellum and by 60.8 % in the temporal cortex of subjects with autism, suggesting glutathione redox imbalance in the brain of individuals with autism (Chauhan et al. 2012a). Such alterations in glutathione levels were not observed in the parietal and occipital cortices of subjects with autism as compared to age-matched control subjects. Our findings on decreased GSH levels in the cerebellum of subjects with autism as compared to age-matched controls have been confirmed by Rose et al. (2012a).
Recently, we also reported impaired activities of glutathione-related enzymes involved in the antioxidant defense, detoxification, GSH regeneration and synthesis of glutathione in the cerebellum of subjects with autism as compared to age-matched controls (Gu et al. 2013a). There was significant reduction in the activities of glutamate cysteine ligase (GCL)—a rate limiting enzyme for GSH synthesis, glutathione S-transferase (GST)—an antioxidant detoxification enzyme, as well as GPx activity in the cerebellum of the subjects with autism as compared to age-matched controls (Gu et al. 2013a) .
20.3.3 Relationship Between Homocysteine, Methionine and Oxidative Stress in Autism
Methionine is a main amino acid in the metabolism of glutathione . Several reports have suggested aberrant metabolism of the methionine cycle in autism. Ali et al. (2011) reported hyperhomocysteinemia, an indicator of impaired folate-dependent methionine metabolism, and increased oxidative stress in a case-control study of Omani children with autism. Hyperhomocysteinemia can cause oxidative stress via a number of mechanisms such as auto-oxidation of homocysteine to form ROS (Heinecke et al. 1987), increased lipid peroxidation (Jones et al. 1994), and reduced production of GPx (Upchurch et al. 1997). Pasca et al. (2006) reported higher levels of total homocysteine in the plasma of autistic children compared to control subjects. In the autistic group, a strong negative correlation was observed between homocysteine levels and GPx activity, suggesting an association between high levels of homocysteine and oxidative stress in autism. A clinical study has reported lower concentrations of methionine, cystathionine and cysteine as well as a decreased ratio of S-adenosylmethionine (SAM)/S-adenosinehomocysteine (SAH), an indicator of decreased methylation capacity in the plasma of children with autism (James et al. 2004). According to the “redox/methylation hypothesis of autism” proposed by Deth et al. (2008), oxidative stress initiated by environmental factors in genetically vulnerable individuals can lead to impaired methylation and neurological deficits. Interestingly, the parents of autistic children shared similar metabolic deficits in methylation capacity and GSH-dependent antioxidant/ detoxification capacity, as observed in autistic children (James et al. 2008) .
20.3.4 Decreased Activities of Antioxidant Enzymes in Autism
Not only the generation of ROS is increased in autism, the activities of antioxidant enzymes are also decreased, thus resulting in oxidative stress in autism . Decreased activity of GPx in erythrocytes and plasma (Yorbik et al. 2002; Pasca et al. 2006), and decreased activities of catalase (Zoroglu et al. 2004) and SOD (Yorbik et al. 2002) in erythrocytes have been reported in autism. We also reported increased oxidative damage and free radical generation, coupled with reduced activities of antioxidant enzymes, in lymphoblastoid cells from autistic subjects compared with age-matched control subjects (Essa et al. 2009). In the cerebellum, we recently reported significantly reduced activity of GPx in autism as compared to age-matched control group (Gu et al. 2013a). In a study of Egyptian children, oxidative stress was found in 88.6 % of autistic children, as revealed by elevated plasma F2-isoprostane and/or reduced GPx levels (Mostafa et al. 2010). In Saudi children with autism, decreased GSH levels and SOD activity were observed in erythrocytes (Al-Gadani et al. 2009). Meguid et al. (2011) reported lower levels of SOD and GPx, and increased lipid peroxidation in the blood samples from autistic children as compared with control subjects. Parellada et al. (2012) reported reduced levels of antioxidant status including non-enzymatic antioxidants (GSH and homocysteine) and antioxidant enzymes (catalase, SOD, GPX) in the blood of subjects with Asperger syndrome. Recently, meta-analysis of antioxidant enzymes in autism were completed by two groups (Frustaci et al. 2012; Main et al. 2012), which showed that GPx activity in erythrocytes was significantly lower in subjects with ASD than that in controls, while the activity of SOD in blood failed to demonstrate any significant association with autism .
20.3.5 Metals and Autism
Trace metals (such as copper and iron) and heavy metals (such as lead, cadmium and mercury) are known to induce oxidative stress . Adams et al. (2013) reported higher levels of lead in erythrocytes, and higher urinary levels of lead, thallium, tin and tungsten in the children with autism, which strongly correlated with severity of autism. In the hair samples of subjects with autism, Al-Farsi et al. (2013) reported higher levels of heavy metals ranging from 150 to 365 % of controls .
Copper and iron play important roles in the redox metabolism, and act as pro-oxidants. Their levels are regulated by two transporting proteins: ceruloplasmin (a copper-transporting protein) and transferrin (an iron-transporting protein). Both of these proteins are antioxidant proteins that are synthesized in several tissues, including brain (Arnaud et al. 1988; Loeffler et al. 1995). Ceruloplasmin inhibits the peroxidation of membrane lipids catalyzed by metal ions, such as iron and copper (Gutteridge 1983). We reported reduced levels of ceruloplasmin and transferrin in the blood of children with autism as compared to their developmentally normal siblings (Chauhan et al. 2004). Most importantly, the levels of ceruloplasmin and transferrin were significantly reduced in children with regressive autism who had lost previously acquired language skills (Chauhan et al. 2004) .
Faber et al (2009) reported that plasma Zn/Cu ratio of 0.608 in children with autism was below the 0.7 % cut-off of the lowest 2.5 % of healthy children. Zinc deficiency, copper toxicity and low Zn/Cu in children with ASDs may indicate altered functioning of metallothionein system. Other preliminary studies have also suggested altered serum Cu/Zn ratios in autism (McGinnis 2004). In other study, Russo and Devito (2011) reported higher levels of Cu and lower levels of Zn in the plasma of subjects with autism as compared with controls, and that zinc therapy could decrease the plasma levels of copper indicating an association between plasma copper levels and autism . Similarly, Lakshmi and Geetha (2011) reported increased levels of copper in the hair and nails of children with autism. In a recent study, Percorelli et al. (2013) observed increased levels of non-protein bound iron in the erythrocytes and plasma of children with autism as compared with controls .
20.3.6 Xanthine Oxidase (XO) and NO (pro-oxidants) in Autism
XO and NO have been implicated in the etiology of autism. XO is an endogenous pro-oxidant enzyme that is involved in the production of superoxide radicals during conversion of xanthine to uric acid (Kellogg and Fridovich 1975). Increased XO activity has been reported in the erythrocytes of autistic subjects (Zoroglu et al. 2004) .
NO is synthesized from L-arginine by nitric oxide synthase (NOS). NO is another toxic free radical that can react with superoxide anion and generate cytotoxic peroxynitrite anions (ONOO·) (Fig. 20.1). NO is known to affect the development and function of the central nervous system. NO has been implicated in neurotransmitter release (Lonart et al. 1992), neurite growth (Hindley et al. 1997), synaptogenesis (Truman et al. 1996), memory and learning (Holscher and Rose 1992), and macrophage-mediated cytotoxicity (Hibbs et al. 1988). Sogut et al. (2003) reported increased NO levels in the RBCs of autistic subjects, and suggested that NOS may be activated in autism. Elevated levels of NO have also been reported in the plasma of autistic subjects (Zoroglu et al. 2003; Sweeten et al. 2004; Tostes et al. 2012) .
20.4 Genetic Variations of Enzymes Involved in Oxidative Stress in Autism
Recent evidence suggests association of gene mutations/deletions, copy number variations, and other genetic abnormalities with autism (Sutcliffe 2008) . Some of the genetic variations can also increase vulnerability to oxidative stress in autism, and are discussed in the following sections:
20.4.1 Nitric Oxide Synthase
Fatemi et al (2000) reported that prenatal viral infection affects brain development via pathological involvement of neuronal NOS (nNOS) expression. Genotyping of single-nucleotide polymorphisms (SNPs) in the NOS-I gene and NOS-IIA gene, and haplotype analysis in 151 Korean ASD trios showed significant evidence for an association between NOS-IIA and ASD (Kim et al. 2009) .
20.4.2 Glyoxalase 1
Glyoxalase 1 (Glo 1) has a critical role in the detoxification of dicarboxylic compounds, thereby reducing the formation of advanced glycation end products.Glo 1 uses GSH as a cofactor to detoxify cytotoxic 2-oxoaldehydes, such as methylglyoxal, which are produced by lipid peroxidation, glycation, and degradation of glycolytic intermediates (Thornalley 2003). While two studies reported a SNP in Glo 1 in autism (Junaid et al. 2004, Sacco et al. 2007), other studies did not find an association between Glo 1 gene and autism (Rehnstrom et al. 2008; Wu et al. 2008). However, in a recent review, the role of Glo 1 and methylglyoxal in behavior has been described (Distler and Palmer 2012) .
20.4.3 Monoamine Oxidase A (MAOA)
This enzyme catalyzes the oxidation of endogenous amine-containing neurotransmitters such as serotonin and norepinephrine (Fitzpatrick 2010) . The role of MAOA in autism is of particular interest because this enzyme affects the levels of serotonin, which are known to be abnormal in some individuals with autism (Hranilovic et al. 2007). An association of the 3-repeat MAOA and a 30-base pair (bp) repeat polymorphism (uVNTR) allele (low activity) with increased severity of autism has been reported (Cohen et al. 2003; Roohi et al. 2009; Cohen et al. 2011). Yoo et al. (2009) also reported preferential transmission of the 3-repeat allele of a MAOA-uVNTR marker in ASDs in a Korean population. Davis et al. (2008) reported an association between MAOA-uVNTR polymorphism and brain growth in autism. Their magnetic resonance imaging (MRI) studies showed an increase in the volume of white matter in the brains of children with autism who had the low-activity, 3-repeat allele compared to those with a high-activity, 4-repeat allele. In a case-control study, Tassone et al. (2011) reported that male children carrying 4 tandem repeats in the promoter region of MAOA gene had a two-fold higher risk of autism . In a recent study, MAO A and MAO A/B knockout mice showed behavioral abnormalities such as social and communication impairments, perseverative and stereotypical responses, and behavioral inflexibility similar to those observed in ASDs (Bortolato et al. 2013) .
20.4.4 Cyclooxygenase (COX)
This enzyme is responsible for the formation of important biological mediators called prostanoids, including prostaglandins, protacyclin, and thromboxane . During inflammation, COX-2 is rapidly induced by growth factors, cytokines, and inflammatory molecules. Recently, Asadabadi et al. (2013) reported that combination of risperidone (antipsychotic drug) and celecoxib (COX-2 inhibitor) was better than only risperidone in treating irritability, social withdrawal and stereotyped behavior of children with autism. Yoo et al. (2008) examined the relationship between ASDs and polymorphism of PTGS2 (the gene encoding COX-2) in 151 Korean family trios including children with autism. They reported a significant association of one intronic SNP (OS2745557) and the GAAA haplotypes with ASDs .
20.4.5 Genes Involved in Glutathione Homeostasis
Polymorphism of genes related to glutathione metabolism has been reported in autism (James et al. 2006; Williams et al. 2007; Ming et al. 2010; Bowers et al. 2011) . Wiliams et al. (2007) reported increased risk of autistic disorder in the children of mothers having glutathione S-transferase P1 haplotype. An association between polyalanine repeat polymorphism in GPx gene (GPx-1) and autism has been observed (Ming et al. 2010). Bowers et al. (2011) examined genetic variations in 42 genes related to GSH metabolism in autism samples obtained from Autism Genetic Resources Exchange (AGRE), and reported 308 SNPs in 318 families. Their analysis showed significant association between cystathione gamma lyase, glutaredoxin, glutaredoxin 3 and autism (Bowers et al. 2011) .
20.4.6 Genes Involved in Folate Metabolism
Folate plays an important role in the metabolism of neurotransmitters, and the synthesis of methionine and thymine . The role of the folate gene polymorphism has also been suggested in autism. Adams et al. (2007) reported that the 19-bp deletion polymorphism of dihydrofolate reductase is a significant risk factor in autism. James et al. (2006) reported differences in allele frequency and/or significant gene-gene interactions for genes encoding the reduced folate carrier and methylene tetrahydrofolate reductase (MTHFR). In other studies, MTHFR C677T was suggested as a risk factor for autism (Mohammad et al. 2009; Guo et al. 2012). Recently, Schmidt et al. (2012) reported association of maternal periconceptional folic acid intake with reduced ASD risk, which was strongest for mothers and children with MTHFR C677T variant genotype. Folate deficiency can increase oxidative stress by increasing the levels of homocysteine. Ali et al. (2011) observed decreased levels of folate and increased levels of homocysteine in the serum of Omani children with autism. Kaluzna-Czaplinska et al. (2011) reported increased urinary excretion of homocysteine in children with autism, thus suggesting a deficiency of folate in blood .
20.5 Role of Mitochondria in Free Radical Generation, and Mitochondrial Dysfunction (MD) in Autism
Free radicals are generated endogenously during oxidative metabolism and energy production by mitochondria in the cell (Cadenas and Davies 2000; Lenaz 2001). In a recent meta-analysis of data in the literature, Rossignol and Frye (2012b) reported the strongest evidence for immune dysregulation/inflammation and oxidative stress, followed by toxicant exposures and mitochondrial dysfunction in autism. Several studies with blood, muscle biopsy, and postmortem brain tissue samples have suggested mitochondrial dysfunction in a subset of individuals with autism (Gargus 2008; Haas 2010; Palmieri and Persico 2010; Chauhan et al. 2011b, 2012b; Gu et al. ; Rossignol and Frye 2012a, b). The review of previous reports and meta-analysis conducted by Rossignol and Frye (2012a) suggested deficiencies of mitochondrial ETC complexes I, III, V, IV, and II in 53, 30, 23, 20 and 9 % of children with ASD and concomitant MD, respectively. Multiple complex deficiencies were reported in 36 % of the children with ASD/MD. In the lymphoblastoid cells from autistic subjects, we reported reduced mitochondrial membrane potential (MMP) and increased free radical generation (Chauhan et al. 2009b). Recently, we also reported brain region–specific changes in the levels of mitochondrial ETC complexes in the subjects with autism (Chauhan et al. 2011b). In autistic children (4–10 years of age), we observed significantly lower levels of complexes III and V in the cerebellum, of complex I in the frontal cortex, and of complexes II, III, and V in the temporal cortex as compared to age-matched control subjects. In the cerebellum and temporal cortex, no overlap was observed in the levels of these complexes between autism and control subjects. In the frontal cortex, decreased levels of ETC complexes were observed in 60 % of autism cases for complexes I, II and V, and in 40 % autism cases for complexes II and IV (Chauhan et al. 2011b).
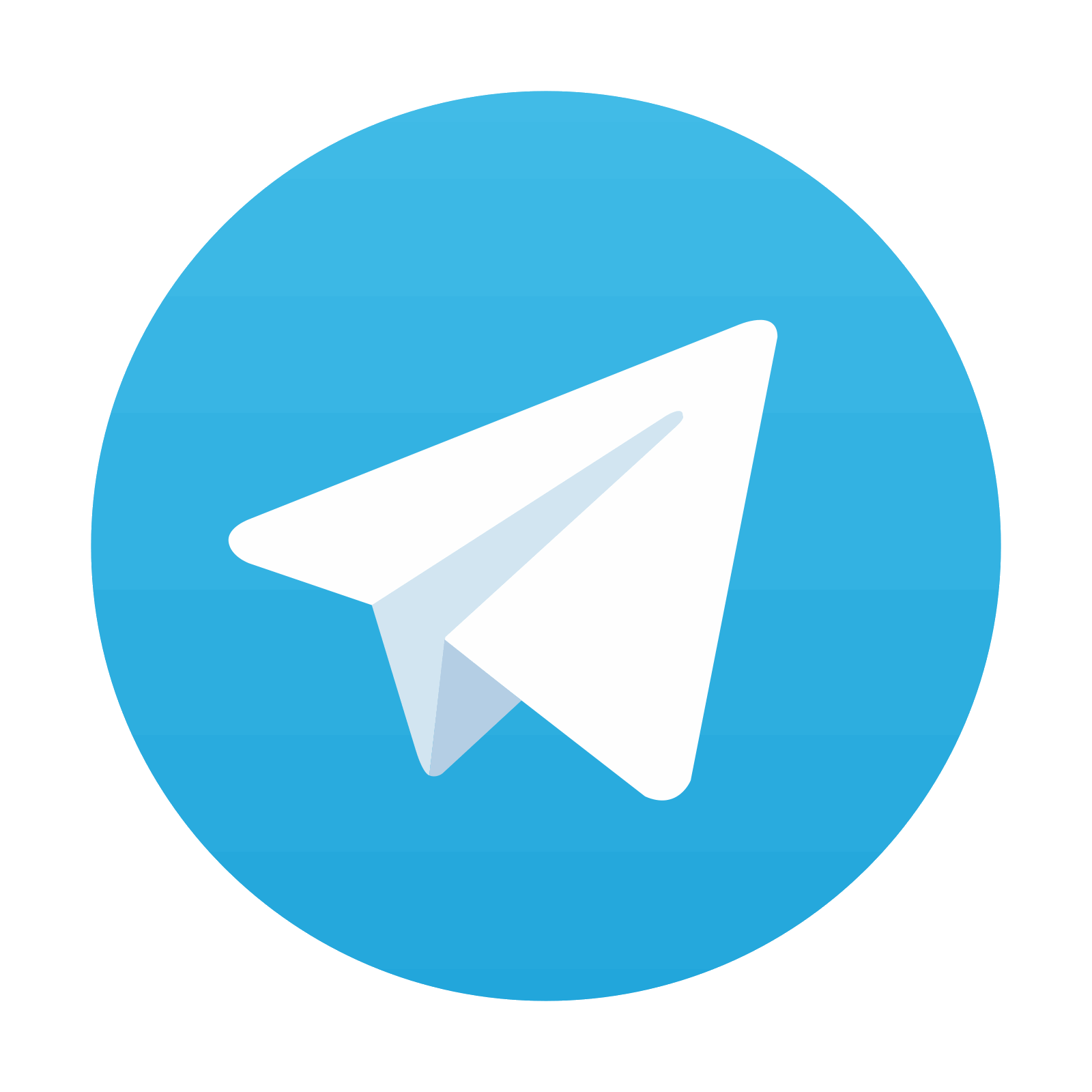
Stay updated, free articles. Join our Telegram channel
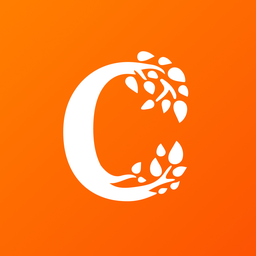
Full access? Get Clinical Tree
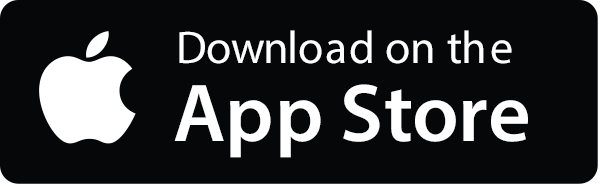
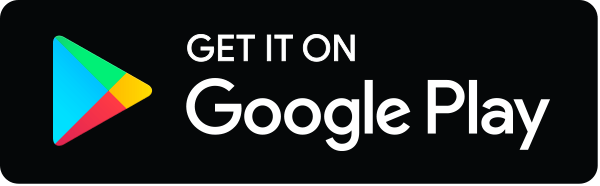