13 Philip A. Barber Calgary Stroke Program; and Departments of Clinical Neurosciences and Radiology, Hotchkiss Brain Institute, University of Calgary, Alberta, Canada Stroke is a highly complex process, involving cerebrovascular and parenchymal tissues through the interaction of multiple mechanisms. In the acute stage, the processes are dominated by calcium-mediated cell demise, activation of lipolytic pathways and alterations in protein phosphorylation related to the depletion of adenosine triphosphate (ATP) stores with depression of glucose metabolism (Siesjo, 1992a,b). These events are modified by the generation of free radicals, specifically nitric oxide generation, protein oxidation and lipid peroxidation (Siesjo, 1992b; Siesjo and Katsura, 1992; Siesjo et al., 1995). At the cellular level, there is an activation of cell-signalling pathways and decreased endothelial cell matrix expression. Associated with this cascade of events are major changes related to the blood–brain barrier (BBB), basal lamina degradation, the detachment of astrocyte end feet and the expression of matrix proteases (Hamann et al., 1996; Haring et al., 1996; Wagner et al., 1997). The combination of pro-inflammatory cytokine and endogenous tissue factors is known to contribute to endothelial cell adhesion receptor activation of polymorphonuclear leukocytes and monocytes, and increased adhesion and transmigration of polymorphonuclear (PMN) leukocytes and platelet activation, accompanied by increasing leakage of the BBB (Giulian et al., 1989; Hallenbeck, 1996). Established tissue injury results in oedema formation, cellular swelling and a focal no reflow within the ischaemic regions and ongoing increase in microvascular permeability with leakage. Following focal stroke, the extent of the brain injury is ultimately dependent on the critical thresholds of reduced cerebral blood flow (CBF), duration of the ischaemic insult, tissue temperature, blood glucose level and other physiological variables (Barber et al., 2004). Flow is dramatically diminished but usually persists at a low level in the microvasculature. Some neurons are thought to be more vulnerable to hypoxia and decreased CBF than other neurons, such as the CA1 cells in the hippocampus; this is termed ‘selective vulnerability’. With prolonged reductions in CBF below 10 ml per 100 g of brain tissue per minute, cellular transport mechanisms and neurotransmitter systems fail, potentially toxic neurotransmitters are released (Rothman and Olney, 1986), free radicals and lipid peroxides are formed which further injure the cells (Kontos et al., 1979; McCord, 1985), and potentially neurotoxic platelet-activating factor (PAF) is released from neurons (Lindsberg et al., 1991). At this threshold, lack of oxygen inhibits the mitochondrial metabolism and activates the inefficient anaerobic metabolism of glucose, causing a local rise in lactate production and so a fall in pH, leading to intra- and extracellular acidosis. The energy-dependent functions of cell membranes to maintain ion homeostasis become progressively impaired. Potassium ions leak out of cells into the extracellular space, Na+ and water enter cells (cytotoxic oedema) and Ca2+ enters the cell, where it impairs mitochondrial function and compromises intracellular membranes to control subsequent ion fluxes, leading to further cytotoxicity. This degree of ischaemia represents a ‘threshold for loss of cellular ion homeostasis’. Mechanisms that give rise to ischaemic cell death occur via three major mediators: unregulated increases of Ca2+ concentration intracellularly, tissue acidosis, and nitric oxide and free radical production. Repeated waves of spreading depression further compromise the reduction in CBF. Additionally, the ischaemic brain injury is modulated by inflammation, by the induction of immediate early genes and later by apoptotic mechanisms (Dirnagl et al., 1999). Extracellular Ca2+ concentrations are several thousand times greater than its intracellular concentrations, and the mechanisms that control this gradient are energy dependent. Calcium enters the cell predominantly through two types of channels: voltage-controlled and receptor-operated channels. There are several agonists that increase permeability to Ca2+ when bound to specific receptors, namely, excitatory amino acids (glutamate, nucleotides and cyclic nucleotides) (Siesjo, 1992a,b). The export of Ca2+ from neurons into the extracellular environment occurs via processes which are linked directly or indirectly to the utilization of energy (Kontos et al., 1979; Lindsberg et al., 1991). In the former category is the ATP-dependent calcium pump, and in the second is the Na+–Ca++ exchanger that utilizes energy stored in the sodium electrochemical gradient. The exchanger can operate in either direction so that when the Na+ gradient falls, it can import rather than export Ca2+. Substantial amounts of Ca2+ are stored within intracellular organelles, mainly the mitochondria and endoplasmic reticulum. Release of Ca2+ from mitochondria requires energy, which is either Na+ dependent or independent. The accumulation of Ca2+ in the endoplasmic reticulum requires energy and is mediated by an ATP-consuming pump (Barber et al., 2004). Calcium enters cells by ionotropic-operated calcium channels, which are predominately gated by glutamate receptors of the N-methyl-D-aspartate (NMDA) type and by voltage-sensitive calcium channels. When glutamate is released from presynaptic endings, it activates two types of ionotropic glutamate receptors, the NMDA type and the non-NMDA type, with the latter being selectively activated by amino-3-hydroxy-5-methyl-4-isoxazolepropionic acid (AMPA). The AMPA receptor (AMPAR) gates a channel that is permeable to monovalent cations (Na+, K+ and H+), while the NMDA-gated channel is also permeable to Ca2+. This channel is blocked by Mg2+, which is released when the cell membrane depolarizes following AMPAR activation. Depolarization also allows Ca2+ to enter via voltage-sensitive calcium channels (Siesjo, 1992a). The decline in CBF and the accompanying loss of oxygen supply result in impaired energy metabolism and measurable decreases in ATP and phosphocreatine. The combination of ATP breakdown and compensatory anaerobic glycolysis leads to cellular acidification. With energy depletion, the membrane potential is lost and neurones and glia depolarize (Katsura et al., 1992). This triggers Ca2+ influx through voltage-sensitive Ca2+ channels which further depolarizes the membrane, and excitatory amino acids are released into the extracellular space. Energy-dependent processes such as glutamate re-uptake are impaired, which further increases extracellular glutamate, resulting in prolonged activation of membrane glutamate receptors and Ca2+ influx (Dirnagl et al., 1999). Sodium and Cl− enter the neuron via channels for monovalent ions (Tyson et al., 1996). Water follows passively, and the ensuing oedema can affect the perfusion of regions surrounding the focally injured brain and have more remote effects that produce increased intracranial pressure and vascular compression. The consequences of the unregulated rise in intracellular cytoplasmic Ca2+ during ischaemia are linked with glutamate excitotoxicity to a number of biochemical processes that result in further detrimental injury to ischaemic brain tissue (Dirnagl et al., 1999). Some of these events continue irrespective of re-oxygenation, while others are provoked or triggered during reperfusion. The activation of lipolysis produces an increase in free fatty acids (FFAs), which are potentially neurotoxic, and the oxidation of arachidonic acid (catalysed by cyclooxygenase and lypooxygenase) yields prostaglandins, leukotrienes and thromboxane A2. These agents, together with PAF, can damage cellular membranes and act additionally as chemoattractants (Dirnagl et al., 1999). The activation also produces oxygen free radicals. These reactions can produce sustained damage to the cell membranes and receptors, further accelerating the toxic effects of glutamate by impaired uptake. Many of the enzymatic processes involved in the phosphorylation of proteins are Ca2+ mediated. An alteration in protein kinase and phosphatase activity during ischaemia provokes changes in ion membrane and receptor activity, and affects gene transcription and translation. The effect of these processes may perhaps explain the long-term effects of ischaemia on membrane function and integrity, facilitating free radical peroxidation as well as unmasking processes leading to programmed cell death (apoptosis). During ischaemia, other Ca2+-mediated reactions are accelerated such as proteolysis, and the assembly and reassembly of microtubules (Baudry and Vicaut, 1993). Cytoskeletal proteins are degraded when Ca2+ rises intracellularly. Several Ca2+-dependent enzymes trigger reactions that produce reactive oxygen species (ROS). These events proceed during ischaemia if there is residual blood flow but are greatly accelerated during reperfusion. Some of the reactions involved result in the oxidation of arachidonic acid (by cyclooxygenase and lipooxygenase), the oxidation of xanthine and hypoxanthine to O2• and H2O2 (via xanthine oxidase) and those reactions leading to the formation of NO• (by nitric oxide synthase). However, the vast majority of ROS are produced by the mitochondrial respiratory chain, and because mitochondria contain superoxide dismutase the result is the formation of O2• and H2O2. Mitochondria have been increasingly implicated in the pathophysiology of ischaemic brain injury. Free radical–mediated disruption of the inner mitochondrial membrane and the oxidation of proteins that mediate electron transport impair their function. The mitochondrial membrane becomes leaky, and eventually mitochondria become overloaded with Ca2+, resulting in impaired ATP production and ultimately energy and membrane failure (Budd and Nicholls, 1996). Cytochrome C is released from the mitochondria and has been suggested as a trigger for apoptosis (Dirnagl et al., 1999). Free radicals are produced in small quantities in cells during aerobic metabolism. Mitochondria are the major producer of free radicals in biological systems. Superoxide (O2•) is formed during the operation of complex I and complex II of the mitochondrial electron transport chain. However, they are extremely toxic, causing damage to DNA, proteins, lipids and components of the extracellular matrix. Polyunsaturated fats and sulphur-containing amino acids that are found in high concentrations within the brain are particularly vulnerable. Free radical scavengers such as tocopherol and vitamin C enzymes, which metabolize free radicals (via superoxide dismutase), help maintain the balance physiologically. During severe ischaemia, insufficient O2 is available to accept electrons passed along the mitochondrial electron transport chain. Free radicals are also generated during ischaemia by the release of iron from ferritin stores within ischaemic neurones (Davalos et al., 1994). As the cerebrospinal fluid has a low concentration of ferritin-binding proteins, much of the iron released from damaged brain cells remains unbound and is therefore available to catalyse the generation of radical hydroxyl (OH•), leading to iron-induced lipid peroxidation. This process is further compounded by the fact that the central nervous system is relatively depleted of superoxide dismutase, which scavenges OH• and controls the release of iron from intracellular stores. The commonest free radicals produced during cerebral ischaemia are O2• and OH•, and these, like other free radicals, react with and exacerbate the damage of proteins, DNA and lipids, particularly the fatty acid component of the cell membrane, causing changes in the permeability and integrity of the cell membranes (lipid peroxidation). It has been shown using salicylate trapping of OH• that brain undergoing ischaemia reperfusion produces oxygen free radicals during the reperfusion phase after ischaemia (Cao et al., 1988). Activated leucocytes produce oxygen free radicals which, during ischaemia, become localized on the brain microvessels through the action of P-selectin, exposing the microvascular endothelium to high levels of oxygen free radicals and causing oxidative damage to specific local sites. This contributes to the breakdown of the BBB and to brain oedema. Focal ischaemia is associated with differentiated cellular responses involving microglia, neurons, astrocytes and endothelial cells (Rothwell, 1997). The immediate inflammatory response is primarily coordinated by the resident microglia. These cells appear to be activated within seconds and minutes of the onset of ischaemia. Their activation promotes the release of the important inflammatory cytokines and chemokines, including tumour necrosis factor alpha (TNFα) and various interleukins. Central to the cellular propagation of the inflammatory response are Toll-like receptors (TLRs); these recognize endogenous interleukins termed damage-associated molecular patterns (DAMPs) (Arslan et al., 2010; Iwata et al., 2010). TLR2 and TLR4 have been specifically shown to be up-regulated in stroke and are essential components of the adaptive immune response following ischaemic injury. Neurons, within seconds and minutes of the onset of ischaemia, depolarize and become overloaded with calcium in a process termed ‘excitotoxicity’. They also produce free radicals, which are involved in DNA damage resulting in cellular necrosis. When injury is less severe, neurons may die by a process called programmed cell death (apoptosis), an energy-dependent process requiring ATP. Astrocytes swell and also depolarize, and their injury disturbs the normal homeostasis and buffer of excitatory amino acids; the end result is a propagation of the excitotoxic injury. The endothelium becomes activated by cytokines which up-regulate adhesion molecules which result in the attraction, adhesion, rolling and then diapedesis of peripheral inflammatory cells such as polymorphonuclear leukocytes. The endothelium is also involved in the matrix metalloproteinase of up-regulation that results in BBB disruption and eventual breakdown which can result in a spectrum of injury: in ascending order, these injuries are paracellular movement of water, protein extravasation and, in the extreme situation, red blood cells that are observed clinically as haemorrhagic transformation. In addition, there is evidence for the production of cytokines by peripheral blood mononuclear cells, including monocytes, T lymphocytes, natural killer cells and PMN leukocytes, which can exacerbate CNS inflammation and gliosis. In support of this, peripheral irradiation or treatment with colchicine attenuates inflammation, wound healing and gliosis (Giulian et al., 1989). The early accumulation of neutrophils in the ischaemic brain has been confirmed histologically (Hallenbeck et al., 1986; Clark et al., 1993; Dereski et al., 1993; Liu et al., 1993) by biochemical studies (Barone et al., 1991, 1995), and in radiolabelled leukocyte studies (Pozzilli et al., 1985; Dutka et al., 1989; Hsu et al., 1993). A rapid transient peripheral neutrophilia precedes brain infiltration (Chapman et al., 2009), and the degree to which this peripheral response has been shown in humans correlates with infarct size (Huang et al., 2006; Buck et al., 2008). Focal ischaemia is a very powerful stimulus to elicit genomic responses in the brain in the form of multiple early gene expression. In addition to the genes expressed for cytokines, TNFα, IL1β and many other inflammatory genes are expressed during focal ischaemia. Depending on the severity of the ischaemia and the intrinsic nature of the neuronal populations, it is thought that a stress response and changes in gene expression are elicited, which may be vital to cell survival and repair. Following focal ischaemia, immediate early genes are the first to be regulated, as shown by the identification of c-fos, c-jun and zinc finger genes, but this expression is only transient (Hsu et al., 1993; Wang et al., 1995). A further phase consists of heat shock protein expression, and increased cytokine gene expression, for TNFα and IL1β but also IL6 (Wang et al., 1995) and IL1 receptor antagonist (IL1ra) (Wang et al., 1997). Chemokines such as IL8 (Liu et al., 1993), CXCL10 (IP10) and CCL2 (MCP1) (Wang et al., 1998) are also elevated, and they play important roles in neutrophil and mononuclear cell infiltration, respectively. Subsequent gene expression involves the transcription of proteolytic enzymes (metalloproteinases (MMPs)), which are implicated in the remodelling of the extracellular matrix (Rosenberg et al., 1996; Romanic et al., 1998; Wang et al., 1998) and their endogenous proteinase inhibitors, tissue inhibitors of metalloproteinases (TIMPs) (Cao et al., 1988). This regenerative phase also involves the transcription of mediators such as transforming growth factor beta (Rosenberg et al., 1996) and osteopontin (Ellison et al., 1998), which appear to be important in tissue remodelling and in creating a barrier to protect the residual intact brain tissue. The post-ischaemic inflammatory response can contribute to secondary brain injury in several ways. Under the influence of inflammatory mediators, the cerebral endothelial microvasculature becomes actively pro-thrombotic (Hallenbeck et al., 1986). The rheologic effects of ‘sticky’ leukocytes in the blood interfere with microvascular perfusion. Platelets, fibrin deposition and PMN leukocytes can cause aggregates within the microvasculature (Dutka et al., 1989. Leukotriene and prostaglandin synthesis may contribute to ischaemic injury by causing vasoconstriction and activating platelets and neutrophils, a process that can cause additional leukocyte activation by a ‘vicious circle’ phenomenon (Nishida and Markey, 1996). Infiltrating neutrophils produce inducible nitric oxide synthase (iNOS), an enzyme that produces toxic amounts of nitric oxide (NO), the pathogenic importance of which is reflected by the observation that pharmacological inhibitors of iNOS attenuate ischaemic damage (del Zoppo and Mabuchi, 2003; Iadecola, 2004). The inflammatory process is also linked to apoptosis. It has been observed that antibodies against adhesion molecules attenuate ischaemic injury and reduce apoptotic cell death (Iadecola and Alexander, 2001). Central to this apoptotic cell death is nuclear factor kappa B (NF-κB), a well-characterized, ubiquitous and inducible transcription factor that plays an important role in inflammation, and also in apoptosis and the cell cycle (Baeuerle and Baltimore, 1996; Carroll et al., 2000). In non-stimulated neuronal cells, the inhibitor (IκB) masks the nuclear transport signal by binding to NF-κB, forming a dimer. Upon activation, IκB is degraded by the proteasome complex, revealing the nuclear localization signal, and NF-κB translocates to the nucleus where it activates the transcription of target genes, including cell adhesion molecules, iNOS, cyclooxygenase-2 (COX2), p53, MnSOD and Bcl2. In vivo, NF-κB is strongly induced in animal models of focal cerebral ischaemia (Coulter et al., 1984; Salminen et al., 1995; Carroll et al., 2000). Different experiments suggest that NF-κB can promote either cell death or survival, depending on the paradigm. Mice lacking the NF-κB subunit p50 have significantly smaller lesions after focal cerebral ischaemia (Schneider et al., 1999). Inhibition of NF-κB by a proteasome inhibitor (CVT-634) also reduces ischaemic damage (Buchan et al., 2000). Both of these experiments support the idea that, overall, NF-κB is detrimental for neuronal survival. On the contrary, the capacity of NF-κB to promote expression of anti-apoptotic genes such as Bcl2 and MnSOD suggests that NF-κB can also promote cell survival (Mattson et al., 1997). Along these lines, overexpression of NF-κB in vitro renders neurons more resistant to glucose deprivation and glutamate excitotoxicity (Yu et al., 1999). NF-κB also promotes expression of NAIP (nuclear apoptosis inhibitor protein), which renders neurons more resistant to ischaemia in vivo (Xu et al., 1997; Stehlik et al., 1998). NF-κB activates iNOS and COX2, and it plays a major role in later stages of inflammatory ischaemic brain injury (Iadecola and Alexander, 2001). The interplay between NF-κB-induced cell survival and death has important implications, and hence limitations, for disease-modifying approaches targeting NF-κB.
Inflammatory Mediators and Dysfunction of the Neurovascular Unit following Ischaemia Reperfusion
Focal ischaemia and early mechanisms of injury
Calcium ion homeostasis
Free radical formation
The ischaemic inflammatory response
The neurovascular unit
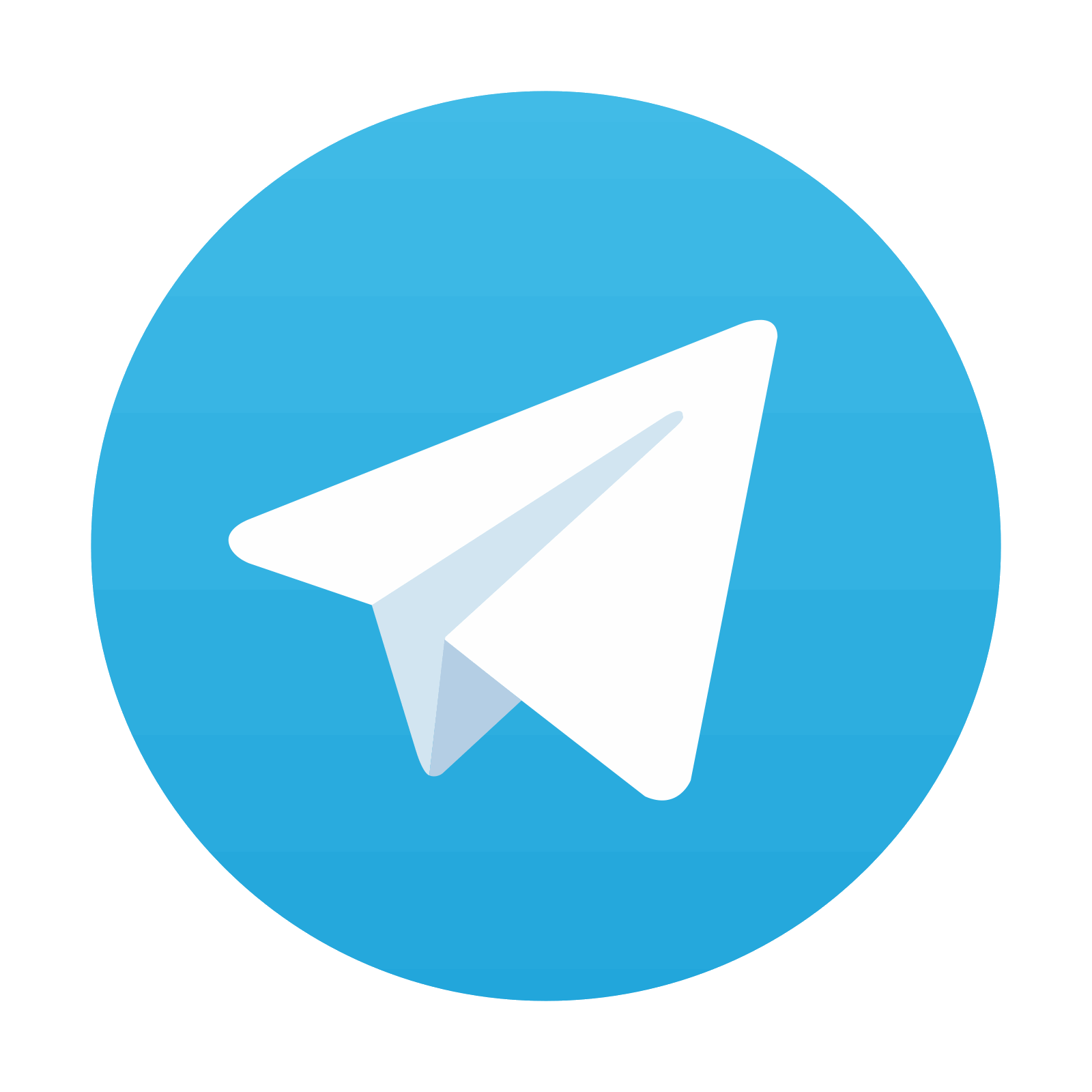
Stay updated, free articles. Join our Telegram channel
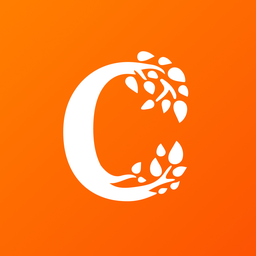
Full access? Get Clinical Tree
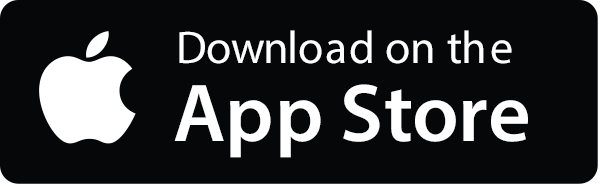
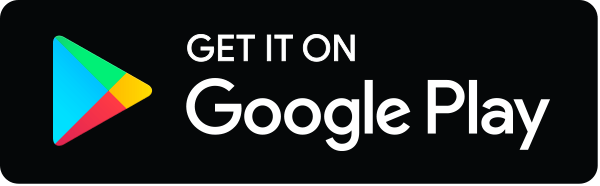