New Orleans criteria
The Canadian head CT rule
Age > 60
Vomiting
Headache
Drug or alcohol intoxication
Persistent anterograde amnesia
Visible trauma above the clavicle
Seizure
High risk for neurosurgical intervention:
Age > 65
Two or more episodes of vomiting
GCS < 15 at 2 h after injury
Suspected open or depressed skull fracture
Any sign of basal skull fracture
Medium risk for brain injury detection by CT:
Retrograde amnesia > 30 min
Dangerous mechanism (pedestrian struck by a motor vehicle, occupant ejected from a motor vehicle, fall from a height of at least 3 ft or 5 stairs)
Imaging Findings
CT is the modality of choice for rapid evaluation of the head. It allows for easy identification of both extraaxial (epidural, subdural, subarachnoid, intraventricular) and intraaxial (surface contusion, intraparenchymal hematoma, shear injury) hemorrhages. An intracranial hemorrhage is seen as an increased density on the CT thanks to its highly proteinaceous nature. There is a linear relationship between the attenuation observed on the CT and hematocrit concentration. The density of the whole blood (hematocrit of 45 %) is about 56 HU. The attenuation of normal gray matter is about 37–41 HU, whereas the density of the white matter is about 30–34 HU [9]. These properties make blood usually easily identifiable on a CT. CT, however, has its limitations. In patients who are severely anemic blood may not be sufficiently dense to be detected. Small hemorrhages near bone are subject to volume averaging artifact. This is particularly common along the floor of the anterior and middle cranial fossae as well as near the convexities. In such cases reformatted coronal, and if needed, sagittal images are very helpful. Blood may be obscured by beam hardening artifact related to dense bones, radiopaque foreign bodies, or support structures outside of the patient. Acute blood gradually decreases in density becoming isodense in subacute stage (8 days–1 month) and hypodense in chronic stages (over 1 month) [9]. Small amounts of subarachnoid hemorrhage may become diluted and inapparent after 1 or 2 days.
An epidural hematoma usually occurs at the coup site. It can result from an injury to a meningeal artery or vein, diploic vein, or a dural venous sinus. It is usually associated with a fracture of the adjacent skull. It is classically lentiform in shape located between the dura and the skull. Epidural hematomas do not cross the suture lines as the periosteal layer of the dura adheres closely to the suture. They, however, can cross midline (underneath the falx cerebri) or between the supratentorial and infratentorial compartments (behind the tentorium cerebelli) as the periosteal layer of the dura forms the outer wall of the adjacent major dural venous sinus which is displaced by the hematoma from the inner table of the skull. Epidural hematomas are usually uniformly dense, however, can contain heterogeneous areas of lower density in cases of rapid bleeding or coagulopathy.
A subdural hematoma occurs at the contrecoup and slightly less commonly the coup site. It represents blood accumulation between the meningeal layer of the dura and the arachnoid. It is caused by injury to the superficial bridging veins. It is crescentic in shape, can cross the suture lines, however, unlike the epidural hematoma, does not cross underneath the falx cerebri or the tentorium cerebelli. The subdural hematomas are usually homogenously dense, and when small, may occasionally be difficult to separate from the adjacent bone. Evaluation with subdural CT window is usually helpful. They may be lesser or mixed density in cases of rapid bleeding, particularly in coagulopathic patients, in patients who are anemic or in cases of acute on chronic subdural hematomas. A rare, but potentially confusing mixed density subdural hematoma can be seen in cases of an arachnoid tear resulting in cerebrospinal fluid mixing with subdural blood or in cases of a subdural hematoma extending into an arachnoid cyst.
Subarachnoid hemorrhage can result from tears of small arteries and veins along the surface of the brain in the subarachnoid space, blood extension from a cortical contusion, or blood extension from the ventricular system through the foramina of Luschka and Magendie. On a CT, an acute subarachnoid hemorrhage has an appearance of linear densities in the sulci and basilar cisterns. It is frequently located near the convexities or near the areas of parenchymal contusions. Redistributed subarachnoid hemorrhage can often be found in the interpeduncular cistern.
Cerebral contusions occur on either the coup or the contrecoup side and result from brain striking the irregular inner surface of the skull during acceleration-deceleration injuries. They are commonly seen in the low anterior frontal and anterior and lateral temporal regions. They can be hemorrhagic or non hemorrhagic. When non hemorrhagic, they appear as areas of low density. Hemorrhagic components result in foci of density within a larger area of hypodensity. In areas of a more severe injury, small hemorrhages may coalesce to form a larger intraparenchymal hematoma. Most contusions increase in size during the initial 12–24 h and it is typical for them to become more hemorrhagic. In one study just 84 % of traumatic intracranial hematomas reached maximal size by 12 h [10].
Diffuse axonal injury , also called shear injury, represents strain of the axonal cytoskeleton due to acceleration/deceleration and rotational injuries. Disruption of axons occurs not only during the actual trauma, but also during the subsequent days, weeks and even years due to a cascade of biochemical events and Wallerian degeneration [11]. Typically milder injuries involve the parasagittal white matter near the grey-white junction, particularly in the frontal lobes. More severe injury also involves the corpus callosum. Severe injuries, particularly related to rotational forces, also affect the brainstem, mainly the dorsolateral midbrain. Shear injury is thought to be responsible for most of the global cognitive impairment seen in severe head trauma patients.
On a head CT scan diffuse axonal injury is seen as punctate hemorrhages at the lobar gray-white junction, in the corpus callosum and brainstem (Fig. 8.1a). CT, however, is not sensitive as the injury is to a large degree microscopic [12] and only up to 20 % of lesions are hemorrhagic.


Fig. 8.1
DAI in a 63-year-old trauma victim. a Head CT shows small linear hemorrhage in the corpus callosum consistent with hemorrhagic focus of shear injury (long white arrow). Small amount of subarachnoid hemorrhage is seen along the surface of the brain on the right (short black arrow). b Diffusion weighted sequence shows additional focus of nonhemorrhagic injury (white arrow) involving the brainstem. c T2*-weighted gradient echo image confirms findings seen on the CT and reveals an additional small focus of hemorrhagic shear injury (short white arrow). d Foci of hemorrhagic DAI are more apparent on the susceptibility weighted sequence (white arrows). Several foci visible on that sequence (black arrows) are not visible on the T2*-weighted gradient echo images (c)
Secondary effects of trauma develop as a result of several factors, chiefly cerebrovascular flow dysregulation, excitotoxicity, oxidative stress related to free radical formation, energy failure and inflammation. Several of these factors result in brain swelling which is thought to be directly caused by both hyperemia and cerebral edema [13]. Studies demonstrating decreased blood volume following trauma suggest the cerebral edema may the major component of brain swelling. It is thought that the edema is initially mainly vasogenic with cytotoxic edema becoming more prominent over the subsequent hours and days [14]. On CT, cerebral swelling related to hyperemia is seen as effacement of the sulci, the basilar cisterns, and the ventricular margins. Areas of vasogenic edema are low attenuation, while cytotoxic edema results in loss of grey white differentiation. Severe brain swelling leads to increased intracranial pressure and decreased cerebral perfusion pressure which can result in an infarction. Children and young adults are particularly susceptible to post traumatic cerebrovascular flow dysregulation with incidence of brain swelling being nearly twofold higher in children than adults [15].
Brain swelling can lead to brain herniation. A subfalcine herniation occurs when the cingulate gyrus herniates under the falx cerebri. An uncal herniation represents herniation of the medial temporal lobe through the tentorial incisura. A compression of the oculomotor nerve by the displaced temporal lobe can present as “blown pupil”. A direct central transtentorial herniation results from diencephalon and midbrain being forced through the tentorial incisura. Mass effect in the posterior cranial fossa can result in upward transtentorial herniation in which the cerebellar tissue herniates through the tentorial incisura, or in cerebellar tonsillar herniation which occurs when the cerebellar tonsils extend down through the foramen magnum.
Decreased cerebral perfusion pressure can lead to cerebral ischemia. Additionally, ischemia can also be caused by vasospasm, vascular injury in the neck, and mechanical compression of blood vessels related to brain herniation. Infarction in the anterior cerebral artery territory is typical following subfalcine herniation, while infarction in the posterior cerebral artery territory is frequently seen as a result of an uncal herniation. CT usually demonstrates hypodensity in the territory of the affected vessel.
CT is the imaging modality of choice for detection of cranial fractures. They are usually readily identified as linear lucencies extending through the calvarium. Non-displaced fractures which are oriented in an axial plane may be difficult to detect without reformatted multiplanar images or 3D rendering. Fractures extending to the paranasal sinuses, the mastoid air cells, or the middle ear cavity may allow air to enter the intracranial compartment. Pneumocephalus usually resolves over time, but persistent pneumocephalus raises possibility of a CSF leak.
Role of MRI
A typical MRI scan of the brain in a trauma patient includes T1-weighted, T2-weighted, fluid attenuated inversion recovery (FLAIR), diffusion weighted (DWI) and gradient echo T2*-weighted or susceptibility weighted (SWI) sequences. Although contusions frequently demonstrate enhancement, contrast is usually not needed, as it does not increase the conspicuity of lesions related to an acute brain injury. MRI is more sensitive than CT for detecting extra-axial hematomas, both hemorrhagic and nonhemorrhagic contusions, and foci of diffuse axonal injury .
Epidural and subdural hematomas have a variable appearance on MRI depending on the age of the hemorrhage and the amount of protein. In addition, arachnoid tears, areas of rapid bleeding, and presence of an underlying chronic extraaxial hematoma contribute to a heterogeneous appearance of subdural and epidural hematomas. For that reason MRI may not be reliable in determining the age of an extraaxial hematoma. They are, however, readily identifiable on MRI due to intrinsic high soft tissue resolution and multiplanar capabilities of MRI. In a study where 62 consecutive patients with moderate or severe head injury were imaged in an acute phase with CT and MRI, MRI revealed SDHs in 37.1 % of cases while CT in only 6.5 % [16].
MRI is also more sensitive than CT for detection of an acute SAH and intraventricular hemorrhages. FLAIR and SWI sequences are particularly useful with SAH usually seen as linear increased signal intensity on a FLAIR sequence and linear decreased signal intensity on a gradient echo T2* weighted or SWI sequence. The sensitivities of both sequences for detection of SAH vary in literature, but both have been reported to be more sensitive than CT [17, 18] with modern FLAIR sequence performing better than the GRE T2* weighted sequence.
MRI is very sensitive for detection of contusions. They appear as areas of parenchymal T2 prolongation and frequently demonstrate increased signal intensity on the diffusion weighted images. The hemorrhagic component is seen as foci of low signal intensity on the gradient echo and susceptibility weighted sequences.
MRI is significantly better than CT in demonstrating shear injury lesions. The nonhemorrhagic lesions are best seen as small foci of increased signal intensity at the lobar gray-white junction, in the corpus callosum and brainstem on the FLAIR and DWI sequences (Fig. 8.1b). In a study comparing FLAIR, T2-weighted, DWI and T2* weighted gradient echo sequences [19] DWI sequence detected the most lesions showing particular robustness for nonhemorrhagic lesions. Most DWI positive lesions (65 %) showed restricted diffusion. In that study DWI was, however, less sensitive for the detection of hemorrhagic lesions than the T2* sequence. Small bleeds are well delineated on the gradient echo and susceptibility weighted sequences (Fig. 8.1c, d). They are particularly well seen on the newer 3 T scanners which can reveal nearly twice as many lesions as the 1.5 T scanners [20] (Fig. 8.1d). DWI is very useful in detecting the secondary effects of trauma such as an acute infarction.
MRI has clear limitations in an acute trauma setting. It is not always readily available and may not be compatible with support apparatus and metallic foreign bodies. The patients are at risk of airway dislodgment during transport and are at risk for hemodynamic instability during long scan times. The images are frequently degraded by motion. Despite its sensitivity for detection of additional injuries, acute MRI rarely offers additional information that would alter the medical or surgical management. In a study by Manolakaki in which MRI was performed within 48 h of admission [21], one third of MRI studies detected additional findings or described the lesions better than the initial CT. The findings, however, were not clinically significant and did not lead to a change in management.
MRI may, however, play a role in patients in whom minimizing ionizing radiation is desired, for example pregnant or pediatric patients. It can also help in evaluation of patients who have a normal brain CT but demonstrate persistent abnormal neurological findings on the examination.
Follow Up Imaging
Widespread availability of CT has led to its increased utilization in a follow up of trauma patients. The compelling argument for a repeat brain CT for stable patients with brain injury is based on the premise that it could allow for identifications of patients who would benefit from early medical or surgical intervention to minimize secondary brain injury. The counterargument is an increased cost, risks related to transferring the patients within the hospital, and exposure to a potentially unnecessary radiation. The challenge is to utilize a repeat head CT enough to detect progressive injury before symptoms develop, yet without overutilization in patients at low risk of progressive injury.
Multiple studies attempted to define a subset of patients for whom a scheduled repeat head CT would be beneficial. In patients with mild head injury (GCS 13–15) and no neurological deterioration, routine follow up head CT is not indicated [22]. Wang et al. in a systematic review of literature [23] found that in 30 studies they reviewed, progression of injury was shown on a follow up CT in 36 % of trauma patients. A neurosurgical intervention following a repeat head CT occurred in 11 % of patients with 6 % of patients undergoing an surgical intervention based on the results of a head CT. They found that 32 % of patients with progression on CT underwent a change in medical management compared with 4 % without progression on a head CT. The review was hampered by differences in studied populations, inconsistencies in definitions of progression of CT findings and the neurosurgical intervention. They found that coagulopathy and overall injury severity were the most commonly reported risk factors for progression of injury on a CT and were also associated with the need for a neurosurgical intervention.
Subsequent studies further characterized subgroups that benefit from follow up imaging. Park et al. investigated the need for a repeat brain CT in patients with traumatic intracranial hemorrhage [24]. He found that patients with progression of lesions on a CT had lower initial GCS score (mean 14.6 in stable group vs. mean 11.9 in group demonstrating progression) and were more likely to be males. Among various types of intracranial hemorrhages , patients with epidural hematomas, intraventricular hematomas, and multiple lesions were more likely to have progression on the follow up CT (59, 80, and 77 % respectively). In that study no patients with stable scans required neurosurgical intervention and 47 % of patients with worsening scans required intervention. More importantly, of the patients who showed radiological worsening on the repeat head CT, 37 % underwent neurosurgical intervention despite lack of significant neurological deterioration. In a separate study Carlos et al. concluded that routine follow up head CT is indicated in patients with GCS ≤ 8 [25] as results may lead to intervention before neurological deterioration. In addition to low GCS, coagulopathy, male sex, epidural hematoma, multiple intracranial lesions, other risk factors for progression of findings include hypotension and elevated intracranial pressure [26].
Repeat head CT is also indicated in patients who are deeply sedated limiting physical examination. Wurmb et al. found worsening of intracranial pathology on a follow up CT in 54 % of deeply sedated polytrauma patients on mechanical ventilation [27]. In 54 % of these patient the therapy was changed due to results on a follow up CT.
The optimal timing of a follow up head CT varies with the type and extent of injury. Sullivan et al. found that enlargement of epidural hematomas occurred early, detected on average within 8 h of injury and within 5 h of initial diagnosis [28]. Other traumatic intracranial hematomas, however, evolve slower. Yamaki found that just 84 % of traumatic intracranial hematomas reached maximal size by 12 h [10]. In a pediatric population deterioration tends to be caused by cerebral edema which mostly develops over the 48 h after head injury. Timing of the follow up head CT thus needs to be individualized.
A routine head CT in critically ill patients are not without risks with complications occurring in 17 % of patients in one study [29]. The most common complications are hemodynamic instability and dislodgement of the airway. Ionizing radiation related to scans in childhood has been linked to future cognitive effects in adults as well as increased incidence of leukemia and brain tumors [30].
While CT is useful for diagnosis and prognostication of outcome in patients with hemorrhagic injuries, there is a subset of patients with normal or near normal head CTs who fare poorly exhibiting profound cognitive impairment frequently remaining comatose long time after the initial injury. These patients usually demonstrate findings of shear injury on MRI. Areas of shear injury are well visualized on T2*-weighted gradient echo images which are sensitive to local field inhogeneity related to presence of blood products. SWI is a newer variant on a gradient echo sequence in which both the magnitude component of the T2* data and the phase of the MR signal are utilized to increase sensitivity for detection of microhemorrhages. It is able to detect four to sixfold more hemorrhagic shear injury lesions than the standard gradient echo sequence [31]. In addition, the phase images of the SWI can differentiate between diamagnetic and paramagnetic susceptibility effects of calcium and blood, respectively [32].
Since shear injury occurs to a large degree at a microscopic level, it is not fully depicted with conventional MRI techniques. The integrity of the white matter can be better evaluated with diffusion tensor imaging (DTI). This MRI technique characterizes directionality of water diffusion by assessing diffusion in at least 6, frequently 25–30 directions. Within a coherently organized white matter, water diffuses preferentially along the direction of white matter fibers, which is known as diffusion anisotropy and is quantified by a DTI measure called fractional anisotropy (FA). In patients with head trauma the microstructural integrity of the white matter tracts is impaired with resulting decreased FA values [33]. Abnormalities of FA are commonly analyzed by two different methods. In a voxelwise statistical analysis the DTI scan is spatially warped in a way that its white matter tracts coincide with those of a 3D white matter atlas or “mean FA skeleton”. Multiple voxels are analyzed without a priori assumptions. In the region of interest method, specific white matter tracts are a priori designated and DTI derived values such as FA can be calculated in these areas. DTI studies have shown that in patients with brain trauma, injury commonly occurs in frontal association pathways, in particular the anterior corona radiata, uncinate fasciculus, the genu of the corpus callosum, and the inferior longitudinal fasciculus [34]. The extent of microstructural damage as evaluated by DTI in the above mentioned regions which may frequently appear normal on conventional MR imaging correlates with impaired cognitive function [34]. Both methods, however, are best at demonstrating group differences between patients with traumatic head injury and controls and are not practical at this point for evaluation of individual patients.
Functional MRI (fMRI) allows for detection of areas of increased neuronal activity. It is based on blood oxygen level dependent (BOLD) effect. Since the neurons do not have substantial energy stores, increased neuronal activation leads to increased blood flow and increased oxygen extraction. Increase in paramagnetic deoxyhemoglobin should theoretically result in decreased signal on the T2* weighted sequence, however, since there is disproportionate increase in inflowing diamagnetic oxyhemoglobin the net result is an increase in BOLD signal [35]. This allows for creation of activation maps, which are usually superimposed on structural brain images . There has been recent interest if fMRI can help better assess the state of consciousness of patients who seem to be minimally conscious or in a persistent vegetative state. Monti et al. performed fMRI on 54 patients who were either in vegetative or minimally conscious state [36]. They found willful modulation of brain activity in 5 patients, all victims of a traumatic brain injury, 4 of whom were thought to be in a persistent vegetative state based on clinical evaluation. In one case they were able to elicit yes and no answers to questions utilizing their fMRI technique. If further studies confirm these promising early results, fMRI may become an adjunct to clinical evaluation that may help in classifying the state of consciousness of trauma patients with severe brain injury. It could perhaps someday be useful in establishing basic communication with some patients who appear unresponsive.
Outcome Prediction
Compression of basilar cisterns, presence of subarachnoid hemorrhage, midline shift, traumatic intracranial hemorrhagic lesions are predictors of poor outcome [37]. Due to sensitivity of CT for detection of such findings multiple researchers investigated its potential for prediction of outcome. One of the early, widely recognized systems is Marshall classification. It utilizes the status of the basilar cisterns, the degree of midline shift, and the presence or absence of high density lesions to define 4 types of diffuse head injury. Additional 2 diagnostic categories depend on presence of an evacuated or nonevacuated mass lesion. Patients can be classified into one of these categories and their mortality at discharge can be predicted [38]. The system, however, has limitations in classifying patients with multiple injury types and is nonlinear thus limiting its application to use as a severity scale. Maas et al. developed refined classification system [39] which relies on status of the basilar cisterns, presence of midline shift, intraventricular or subarachnoid hemorrhage, or an epidural mass lesion. This system, called Rotterdam score (Table 8.2), is well standardized, validated, and permits a more clear differentiation of a prognostic risk, particularly in patients with mass lesions.
Table 8.2
Rotterdam score for the probability of mortality in patients with traumatic brain injury according to their CT characteristics
Predictor | Score |
---|---|
Basal cisterns | |
Normal | 0 |
Compressed | 1 |
Absent | 2 |
Midline shift | |
No shift of shift ≤ 5 mm Shift > 5 mm | 0 1 |
Epidural mass lesion | |
Present | 0 |
Absent | 1 |
Intraventricular or subarachnoid hemorrhage | |
Absent | 0 |
Present | 1 |
Sum score (add 1 to the score) | +1 |
Brain Death
Diagnosis of brain death allows for withdrawal of care and potential organ donation. Although there is some worldwide variability in required observation period, training and number of required evaluating physicians, there is general agreement on clinical evaluation to asses for cessation of brain function. Occasionally, however, the clinical examination is not reliable or apnea test has to be interrupted due to developing hypotension. Sometimes confirmatory tests are required by local guidelines [40]. In such cases brain death can be confirmed utilizing electroencephalography or somatosensory evoked potentials to demonstrate absent electrical brain activity. Alternatively, imaging can be performed to demonstrate absence of cerebral blood flow. Both digital subtraction angiography (DSA) and cerebral scintigraphy are well established for that determination. DSA is, however, invasive and both modalities are often not practical.
CTA has been proposed as an accurate modality for confirming brain death [41]. Multiple studies, however, have shown that the sensitivity and specificity of CTA is dependent on which vessels are evaluated. In contrast to DSA where contrast opacification is imaged in real time, in most cases, CTA is performed after a set delay following contrast injection. Opacification of the arteries can be seen in brain dead patients due to stasis filling, dilution of contrast into more distal vessels, and due to better sensitivity of CT for detection of contrast compared with DSA [42]. Several scales have been proposed depending which vessels are evaluated for opacification. The most common are 10 point, 7 point, and 4 point scales [43]. The 4 point scale which evaluates the bilateral M4 middle cerebral artery branches and the internal cerebral veins (lack of opacification of all 4 vessels being the positive result) seems the most sensitive reaching a sensitivity of 85 % in one meta-analysis [44]. The specificity of this and other scales is, however, not well established as most studies do not include patients who are not brain dead [44, 45]. CTA, in addition, requires iodinated contrast which is potentially nephrotoxic to the kidneys that could be donated. Although CTA can be used with caution in select cases when its sensitivity and uncertain specificity are taken into account, it cannot be recommended as a standard confirmatory test in evaluation for brain death at this point.
Imaging of Blunt Cerebrovascular Injuries
Indications for Screening
Blunt cerebrovascular injury (BCVI) is defined as damage to extracranial or intracranial cerebrovascular structures resulting from blunt trauma. Injury to the neck vessels is significantly more common than the injury to the intracranial vessels [46]. Historically, injury to the neck vessels was considered rare with early studies quoting 0.08 % incidence among blunt trauma victims [47]. As more aggressive screening became prevalent it became apparent that BCVI is frequently occult and more common, with newer studies quoting up to 1.6 % incidence among the blunt trauma patients [48]. The incidence is even higher in severely injured patients with Mutze reporting an incidence of 2.7 % in patients with Injury Severity Score > 16 [49]. BCVI is significantly more common in adults than in children [50] with reported incidence of 0.03–0.3 % [50, 51] in pediatric blunt trauma victims. Injury to the carotid arteries is more common than to the vertebral arteries. Injury occurs to more than one cervical internal carotid artery in 18–38 % of cases [46]. Injury to both vertebral arteries has been found to occur in 28 % of cases [52] in one study.
Stroke is a dreaded complication of BCVI. The stroke rates related to BCVI vary in the literature with Eastman et al. [53] reporting a representative rate of 15.2 % which decreased to 3.8 % after a change in screening and modification of treatment strategies. Stroke rates as high as 67 % have, however, been reported [54]. BCVI may result in stroke by several mechanisms. Vessel injury results in damage to the intima and frequently the deeper layers of the vessel wall. Thrombus formation near the exposed collagen or in a pseudoaneurysm [55] may lead to a thromboembolic stroke. Alternatively, brain ischemia can be caused by vessel occlusion or severe stenosis related to large thrombus formation, or a blood column dissecting into the vessel wall.
Neurologic sequelae of BCVI are frequently delayed and potentially preventable with treatment. Although it has been suggested that screening of asymptomatic patients may be futile [56], most experts agree that screening during the asymptomatic period before the stroke onset is critical, as stroke rates are lower in treated patients and screening has been found to be cost effective [57, 58].
Extracranial internal carotid artery dissections most commonly occur in their distal cervical portions due to neck hyperextension and rotation with resulting stretching of the carotid arteries over the lateral masses of C1–C3. Neck hypeflexion may result in compression of the carotid arteries between the mandible and the cervical spine. Vertebral artery dissections occur most commonly as a result of cervical spine fractures extending to the foramina transversaria or due to subluxations and frequently involve the V2 and V3 segments.
Based on work performed to a large degree by investigators from Denver and Memphis, high risk criteria for BCVI have been identified (Table 8.3) [59, 60]. Some of the most important include cervical and skull base fractures, severe facial fractures, ischemic stroke, severe head injury, Horner syndrome, and intraoral trauma. Additional studies have demonstrated a 4-fold to 8-fold increase in risk for BCVI in patients with chest trauma. This is particularly true in pediatric population where a non-basilar skull fracture is a significant risk factor as well [51]. An isolated seatbelt sign without other risk factors and with normal physical examination is, however, no longer considered an independent risk factor and should not be used as the sole criterion to elect to screen the patient [61]. The screening protocols have led to a significant increase in detection of BCVI. Nevertheless, it is estimated that more than 20 % of BVCIs occur without any of the established risk factors [62, 63]. Some experts thus advocate more liberal screening which can be accomplished by inclusion of a neck CTA into a whole body CT scan performed for severely traumatized patients.
Table 8.3
Screening criteria for blunt cerebrovascular injury
Denver criteria | Memphis Criteria |
---|---|
Cervical spine fracture | Cervical spine fracture |
Unexplained neurological deficit | Unexplained neurological deficit |
Basilar skull fracture with carotid canal involvement | Basilar skull fracture involving the foramen lacerum |
Le Fort II or III fracture | Le Fort II or III fracture |
Cervical hematoma | Horner syndrome |
Cervical bruit | Neck soft tissue injury |
Stroke on secondary CT | |
Diffuse axonal injury with GCS < 6 | |
Near hanging with anoxic brain injury |
Imaging of BCVI
BCVI grading is based on a scale developed by Biffl et al. (Table 8.4) [64]. The scale was originally developed for grading of carotid injuries based on their appearance on DSA and was rapidly adopted for grading of both carotid and vertebral injuries based on CTA. When applied to the carotid injuries the risk of stroke increases with the injury grade with reported stroke prevalence of 3, 11, 33, 44, 100 % associated with injury grades of I, II, III, IV, V [65] respectively. Grade I lesions are most common with higher grades progressively less common. For vertebral arteries, stroke and mortality rates are not linear with the injury grade. The highest rate of stroke (40 %) is associated with grade II injuries and the lowest rate of stroke (13 %) is associated with grade III injuries.
Table 8.4
BCVI grading scale
Injury grade | Description |
---|---|
I | Luminal irregularity or dissection with < 25 % luminal narrowing |
II | Dissection or intramural hematoma with ≥ 25 % luminal narrowing, intraluminal thrombus, or raised intimal flap |
III | Pseudoaneurysm |
IV | Occlusion |
V | Transection with free extravasation |
BCVI has a wide range of appearances on CTA. Minimal intimal injury has an appearance of mild luminal irregularity which does not result in stenosis. It is best seen on reformatted coronal or sagittal images. It may be difficult or impossible to differentiate from vasospasm [66]. A raised intimal flap has an appearance of a linear filling defect extending from the vessel’s wall into its lumen. It is seen well on axial images (Fig. 8.2b) with reformatted coronal and sagittal images allowing for better characterization of the lesion (Fig. 8.2a). A dissection with intramural hematoma is usually characterized by variable degree of narrowing of the lumen of the vessel. The wall of the vessel is thickened in either circumferential or eccentric pattern due to the hematoma. Occlusions demonstrate lack of enhancement in the lumen of the vessel. Larger vessels, such as the common or internal carotid arteries, frequently demonstrate gradual tapering of the lumen best seen on the multiplanar reformatted images. Smaller vessels, such as the vertebral arteries usually demonstrate more abrupt narrowing. Pseudoaneurysms have an appearance of a focal outpouching extending from the lumen of the artery which may sometimes narrow the native vessel (Fig. 8.2c). Transection with active contrast extravasation appears as a non-contained collection of contrast surrounding the injured vessel. The exact point of vessel disruption may, however. not be easy to identify. Arteriovenous fistulae may also be difficult to recognize. A typical early opacification of the vein may not be apparent on CTA depending on the phase of the contrast bolus. Enlargement of the draining vein may be the only finding.


Fig. 8.2
Vascular injury in a 34-year-old trauma victim. a Curved multiplanar reformatted image demonstrates dissection with marked lumen narrowing. An intimal flap is seen as a thin linear lucency (black arrows). b Source axial image shows the intimal flap as well (black arrow). c Image from DSA performed 24 h later reveals pseudoaneurysm formation (white arrow)
Common pitfalls in CT angiographic evaluation of BCVI include streak artifact related to patient’s dental hardware, spinal hardware, or dense foreign bodies in the neck. Sometimes suboptimal contrast bolus timing, which can be related to patient’s hemodynamic state, can hinder the evaluation for BCVI. Artifact related to gross body motion or swallowing may degrade the images. Atherosclerotic disease and sometimes degenerative spine disease may result in lumen irregularity which may mimic vascular injury. These are, however, usually recognized as such upon more careful review. Congenital variants such as internal carotid artery loops can occasionally mask or mimic vascular injury on the axial images. This is easily rectified by review of multiplanar or 3D rendered images. Congenital hypoplasia or aplasia of the internal carotid artery may be difficult to differentiate from an injury. It is, however, rare and can be recognized by small or absent carotid canal. Asymmetry in caliber of the vertebral arteries is more common and may sometimes present a diagnostic dilemma.
A relatively low prevalence of BCVI calls for a screening modality which is very sensitive and at the same time readily available and inexpensive. DSA is considered a gold standard in evaluation for BCVI. It is, however, an invasive, resource intensive study, not without risks with stroke being a major complication. A 1 % incidence of a transient neurological deficit with 0.5 % incidence of a permanent neurological deficit is commonly quoted [67]. The risk, however, varies widely depending on patient selection and the indication for angiography with a risk of a permanent neurological deficit as low as 0.07 % for some patients [68]. The true risk for trauma patients who are generally young is likely quite low.
Duplex sonography is inexpensive, portable, and noninvasive. It, however, lacks sensitivity which has been reported to be as low as 37.5 % [49]. It is technically limited in evaluation of the frequently injured portions of the neck vessels, such as the distal cervical internal carotid arteries and long segments of the vertebral arteries as they course through the foramina transversaria.
Magnetic resonance angiography allows for simultaneous evaluation of both internal carotid and vertebral arteries. It can be performed without contrast and does not involve ionizing radiation. Fat suppressed T1-weighted images allow direct visualization of an intramural hematoma, and the unenhanced time of flight and contrast enhanced images allow for detection of luminal irregularity, pseudoaneurysm, and vessel occlusion [69]. Diffusion weighted images allow for detection of an early stroke. MRI, however, is usually difficult to perform in an acute trauma setting, particularly in critically ill patients who frequently require extensive support apparatus and orthopedic hardware. Motion artifact can be problematic as findings of vascular injury are often subtle and scan time of the fat suppressed T1-weighted sequence is long. MRI was found to perform moderately well in detection of a traumatic dissection with Biffl et al. reporting a sensitivity of 75 %, specificity of 67 % [70] and Miller et al. reporting a sensitivity of 50 and 47 % and specificity of 100 and 97 % for detection of carotid and vertebral dissections respectively [60]. More recent studies comparing MRA to CTA confirmed superiority of CTA [69].
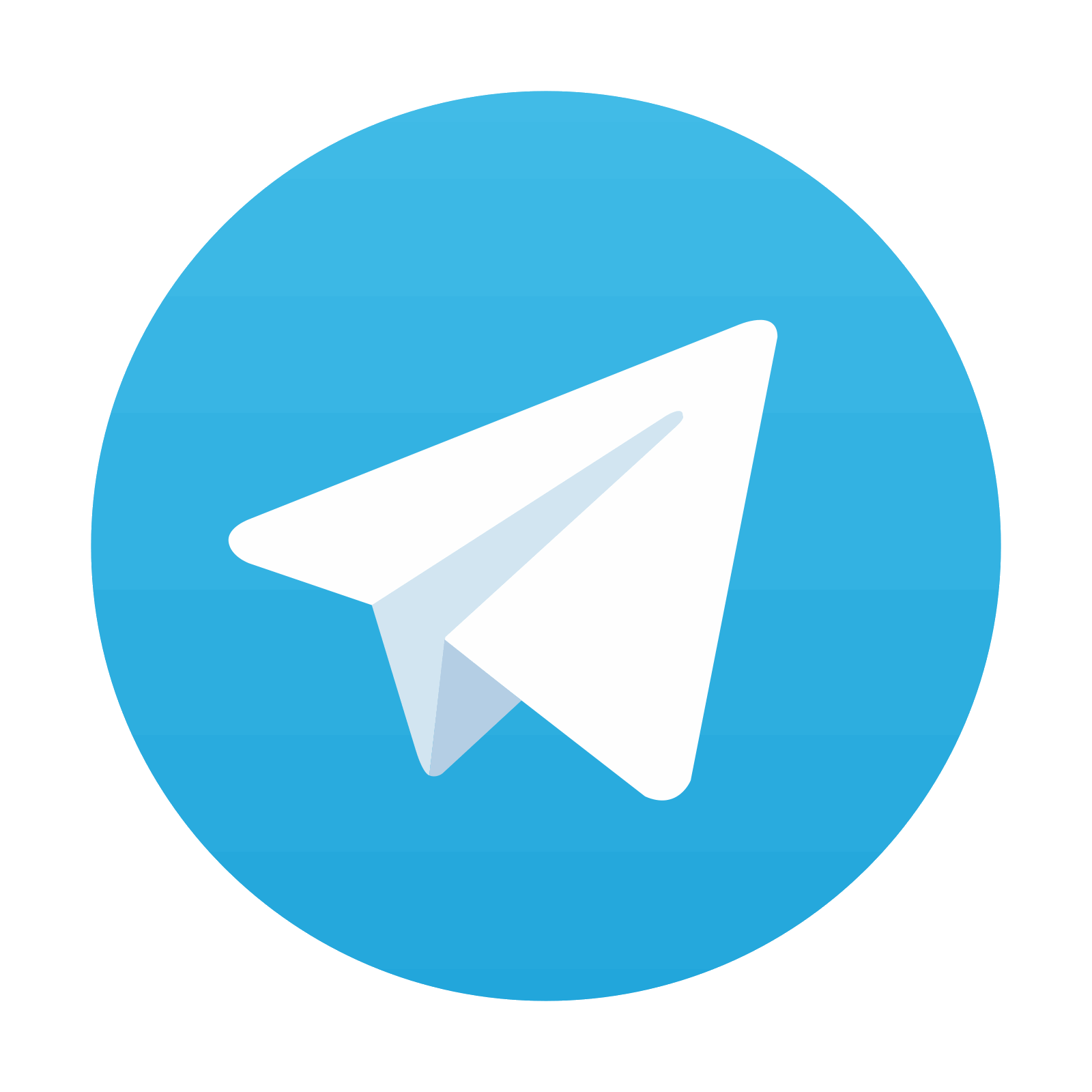
Stay updated, free articles. Join our Telegram channel
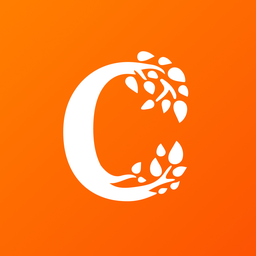
Full access? Get Clinical Tree
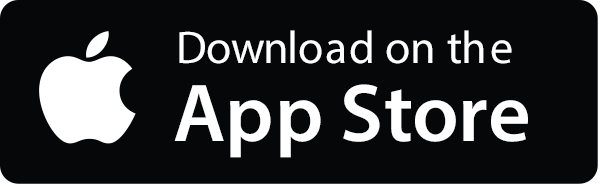
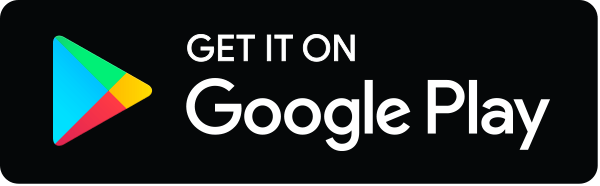