2 Craig S. Moore, Bryce A. Durafourt and Jack P. Antel Department of Neurology and Neurosurgery, Montreal Neurological Institute, McGill University, Montreal, Quebec, Canada The concept of innate immunity is phylogenetically ancient and first appeared in multicellular organisms. Unlike the adaptive immune response, innate immune mechanisms do not rely on ‘memory’ and reset to baseline following clearance of encountered pathogens. The adaptive immune response is dependent on several aspects of innate immunity that result in the generation of antigen-specific lymphocytes and memory cells that are capable of rapidly responding to recurrent infections, thereby providing long-lasting immunity. In order for a pathogen to successfully infect its host, several host defences, including physical barriers, must be breached. These innate barriers include epithelial surfaces and respiratory, reproductive tract and gastrointestinal mucosal surfaces. In the context of the central nervous system (CNS), the blood–brain barrier (BBB) and the blood–cerebrospinal fluid barrier (BCSFB) are considered the most important and relevant barriers; both can be breached during CNS injury. The BBB is both a physical and biochemical barrier, consisting of tight junctions within an endothelial cell barrier that restricts the diffusion of pathogens and large hydrophilic molecules that would otherwise disrupt homeostasis in the CNS. In addition to endothelial cells, astrocytes and pericytes are also integral in forming the BBB and maintaining its integrity by regulating blood flow and providing structural and metabolic support. The BCSFB is located at the tight junctions surrounding the epithelial cells on the surface of the choroid plexus, which secrete cerebrospinal fluid (CSF) and express specific transporter proteins that regulate the exchange of molecules between the blood and the CSF. If a given pathogen is successful and infects its host, an inflammatory response in the host is initiated. While the inflammatory process is very complex, in simple terms, it begins with an innate response whereby local vasodilation of vascularized tissue, including the CNS, leads to fluid and leukocyte accumulation. This increase in blood flow is manifested by symptoms such as swelling (oedema) and redness. Within the blood vessels, increased hydrostatic pressure can lead to the contraction of endothelium and the leakage of fluids, such as collectins (i.e. soluble lectin molecules and mannan-binding protein) and complement, which can bind to the surfaces of microbes in a process termed opsonization, facilitating their phagocytosis or direct lysis. In order for immune cells to reach sites of infection or injury, various molecules and chemoattractants (e.g. bacterial products and chemokines) must promote the expression of adhesion molecules (selectins and integrins) on both immune cells and vascular endothelial cells, while chemokines and activated complement components (e.g. C5a) create a gradient that can lead to extravasation of peripheral blood cells into the infected or injured tissue. Upon reaching the site of injury, phagocytic cells (e.g. neutrophils and macrophages) must identify both opsonized and non-opsonized dying cells, pathogens and cellular debris. The phagocyte can then deliver antimicrobial molecules, engulf the elements and, if necessary, kill the microbe through production of reactive oxygen species (ROS). The detailed mechanisms by which innate immune cells recognize microbes and sites of tissue injury will be discussed in subsequent sections of this chapter. All cells of the immune system, in addition to erythrocytes and platelets, are derived from pluripotent haematopoietic stem cells that develop in the bone marrow. From the bone marrow, leukocytes can either migrate to specialized tissues or reside within the circulatory and/or lymphatic systems. Haematopoietic stem cells can further give rise to both common lymphoid and myeloid progenitors. Cells within the lymphoid lineage differentiate into cells of the adaptive immune system (e.g. B and T lymphocytes) in addition to natural killer (NK) cells. NK cells are large granular cells that lack antigen-specific receptors, but can recognize and kill tumour and pathogen-infected cells in a major histocompatibility complex (MHC) unrestricted manner, and they are therefore considered part of the innate immune system. Common myeloid progenitor cells differentiate into cells of the innate immune system, including granulocytes, mast cells, dendritic cells (DCs) and monocytes and macrophages. Granulocytes are also known as polymorphonuclear cells and can be divided into neutrophils, eosinophils and basophils. During infection and inflammation, these cells can migrate into tissues, where they are found in very large numbers but are relatively short-lived. As stated in this chapter, neutrophils are highly phagocytic and are capable of engulfing and degrading microbes by releasing ROS and hydrolytic enzymes. Of the cells comprising the innate immune system, neutrophils are the most numerous and can readily migrate to sites of injury through various chemotactic gradients. While the roles of eosinophils and basophils have been largely described in the context of allergy, eosinophils in particular have been shown to also possess antiparasitic activity (Rosenberg et al., 2012). In neuromyelitis optica (NMO), an inflammatory demyelinating disease of the CNS, active lesions have been characterized by infiltration of macrophages, perivascular granulocytes and eosinophils (Lucchinetti et al., 2002). Both intact and degranulated eosinophils were found within active lesions; however, their exact function is unknown, and it is unclear whether infiltration of eosinophils is a primary or secondary event in NMO. It has been suggested that complement activation in lesions may result in the production of chemotactic factors that recruit eosinophils into the CNS (Lucchinetti et al., 2002). In addition to their capacity to release ROS, cytokines and growth factors, eosinophils have also been shown to possess antiviral activities through the production of RNases. Mast cells play significant roles in allergy and inflammation, and they are also important in their ability to protect the body from certain pathogens, including parasites (e.g. intestinal worms). While mast cells are capable of secreting a variety of different soluble factors, including histamine, proteases, proteoglycans (heparin), prostaglandins, thromboxanes and leukotrienes, these cells are believed to release several different cytokines that can impact innate immunity, including tumour necrosis factor alpha (TNFα), interleukin-1 (IL1), IL6 and interferon gamma (IFNγ) (Skaper et al., 2012). In the inflamed CNS, mast cells have been found in both multiple sclerosis (MS) and experimental autoimmune encephalomyelitis (EAE) lesions (Brenner et al., 1994; Theoharides, 1990), and can directly cause demyelination and oligodendrocyte death (Johnson et al., 1988; Theoharides et al., 1993; Medic et al., 2010). Much of the current chapter will focus on innate immune cells that are termed professional antigen-presenting cells and include DCs and macro-phages. The CNS resident macrophages, referred to as microglia, will also be discussed in detail. DCs are highly phagocytic cells that possess long extensions resembling the dendrites of neurons. A high density of DCs can be found in epithelial surfaces and in areas that encounter the external environment. Unlike other phagocytic immune cells, DCs specialize in antigen processing and presentation, and effectively present antigen to lymphocytes and initiate adaptive immune responses. Upon antigen uptake, DCs migrate to lymph nodes where they increase their expression of costimulatory molecules, present antigen via MHC proteins and can subsequently activate B cells, naïve T cells and antigen-specific memory T cells that will undergo clonal expansion. It is important to note that both macrophages and B cells can similarly activate T cells; however, the spatial distribution of DCs within the lymph nodes makes them superior antigen-presenting cells and the most potent activators of T cells. In humans, there are two classes of DCs that possess distinct properties that can influence immune responses. These two major cell types are myeloid DCs (mDCs; also termed classical or conventional DCs) and plasmacytoid DCs (pDCs). mDCs are similar to monocytes and are the conventional dendritic cell responsible for activating naïve T cells. There are several different subsets of these specialized DCs; however, all cells express MHC and costimulatory molecules specialized for activating the adaptive immune response (Johnson et al., 2011). While pDCs are also capable of activating T and B cells, they have been documented to play a significant role in responding to viral infections and express specific Toll-like receptors (TLRs) that are able to sense viral infections (TLR7 and TLR9). In addition, pDCs secrete type I interferons (IFNα and IFNβ), which are specialized cytokines that can further activate the immune system and rid the body of viral infections. Monocytes, as well as DCs, also arise from specialized myeloid progenitor cells found in the bone marrow. Monocytes circulate within the blood and are capable of extravasation into tissues to become macrophages. Also included in the myeloid cell category are specialized and tissue-specific macrophages, which have been suggested to arise from the yolk sac (Gomez Perdiguero et al., 2013). Depending on their location within the body, examples of tissue-specific macrophages include alveolar macrophages (lung), Kupffer cells (liver), osteoclasts (bone) and microglia (CNS). Previous fate-mapping studies have demonstrated that adult microglia are derived from haematopoietic progenitors in the extra-embryonic yolk sac between embryonic day 7.0 (E7.0) and E9.0 (Ginhoux et al., 2010). Like DCs, these additional myeloid cell types (e.g. monocytes, macrophages and organ-specific myeloid cells) are phagocytic, express MHC class II and costimulatory molecules and promote adaptive immune responses. However, monocytes and macrophages also secrete a wide array of cytokines and chemokines, which function to promote inflammation and recruit additional immune cells to sites of injury. In subsequent sections of this chapter, the phenotypes and specialized functions of these cells will be further discussed in the context of CNS infection, injury and repair. Prior to activating the adaptive immune response, cells of the innate immune system first respond to pathogens, or danger signals, within their environment. DCs, macrophages and monocytes all possess both surface and intracellular receptors capable of recognizing pathogen-associated molecular patterns (PAMPs), which are small molecular patterns associated with specific classes of pathogens and microorganisms. These specialized patterns are recognized by different families of pattern recognition receptors (PRRs), and they include TLRs, nucleotide-binding oligodimerization domain (NOD)-like receptors (NLRs) and RIG-like receptors (RLGs). The concept of PRRs is important not only in the context of their specialized roles in mediating inflammation, but also in the pathology and identification of specialized and polarized cell types in diseased tissue, as discussed later in this chapter. There are several types of TLRs capable of recognizing different PAMPs, and these can be found both on the cell surface and within endosomes. Common PAMPs and their receptors include glycolipids and lipoproteins (TLR2), double-stranded RNA (dsRNA) (TLR3), lipopolysaccharide (LPS) (TLR4), flagellin (TLR5), single-stranded RNA (ssRNA) (TLR7) and unmethylated CpG DNA (TLR9). A complete table of human TLRs, their respective ligands and their expression within the CNS can be viewed in Table 2.1 (adapted from Hanke and Kielian, 2011). Downstream signalling events of TLR activation are complex, involving several adaptive molecules, kinases and transcription factors. Ultimately, activation of TLRs leads to the transcription of genes that influence inflammatory responses (Athman and Philpott, 2004). Table 2.1 Toll-like receptor (TLR) expression in the central nervous system (Adapted from Hanke and Kielian, 2011.) NLRs are cytosolic receptors and activate similar downstream signalling pathways as TLRs. In mammals, NOD proteins recognize bacterial cell wall proteoglycans and possess CARD domains that can readily recruit caspases, thus activating inflammatory cytokines (e.g. IL1). Mutations in several NOD proteins have been associated with certain inflammatory diseases, including Crohn’s disease (Eckmann and Karin, 2005); however, there are currently no neuroinflammatory diseases identified with such mutations. NLRs are expressed on DCs, macrophages and monocytes, but are also highly expressed in epithelial cells and can activate innate immune responses through downstream signalling. RLGs are also a family of cytoplasmic receptors that contain RNA helicase domains and bind dsRNA, a feature of certain RNA viruses. Downstream signalling of RIG proteins leads to increased expression of type I interferons, important molecules that activate receptors and initiate signalling pathways involved in inhibiting viral replication. PRRs are an important mechanism of infection and injury detection in cells of the innate immune system, and given that expression of these receptors may differ in a pathological context, they may represent novel targets for diagnosis and treatment. Within the CNS, inflammation and innate immunity are believed to contribute to the pathogenesis and neurodegeneration that occur in several diseases and conditions, including stroke, Alzheimer’s disease and demyelinating diseases such as MS. Traditional views that considered the CNS to be an ‘immune-privileged’ site are currently being challenged, whereby it is generally accepted that both innate and adaptive immune responses are important and highly relevant in the context of both CNS injury and repair. In the context of neuroinflammation, while there has been a plethora of research that has investigated the roles of innate immune cells (macrophages, DCs and granulocytes) in contributing to CNS disorders, more recent research has focussed on putative roles of CNS resident cells, including astrocytes and microglia. In the subsequent sections of this chapter, we will discuss the roles of the traditional innate immune cell populations in diseases of the CNS, as well as the emerging roles of CNS resident cells. DCs are among the most important cells in processing antigens and initiating an adaptive immune response. Both in vivo and in vitro, monocytes can give rise to both DCs and macrophages, with each cell lineage being dependent on specific signals that can promote their unique phenotype. Within the non-inflamed or healthy CNS parenchyma, DCs are not present; however, evidence suggests that they can be found in the meninges and the choroid plexus (Anandasabapathy et al., 2011). The highly vascularized nature of these regions suggests that the presence of DCs is likely due to their extravasation from the blood (Prodinger et al., 2011). Following disruption of the BBB, DCs can be found within CNS tissue and readily express surface molecules that are indicative of their activated state (i.e. an increase in costimulatory molecule expression). In EAE, an animal model of MS, studies have indicated that both antigen-specific (memory) and naïve T cells can be activated directly within the CNS by mDCs. Compared to macrophages, pDCs and microglia, mDCs are superior at inducing pro-inflammatory Th17 responses and disease progression (Miller et al., 2007). In EAE, several subtypes of DCs accumulate in the inflamed CNS and are thought to arise from circulating monocytes (Bailey et al., 2007). Within the CNS, DCs have also been shown to be the most important APCs in terms of promoting epitope spreading, a mechanism by which autoreactive T cells can be activated by de novo antigens that are released as a result of ongoing tissue damage (McMahon et al., 2005; Miller et al., 2007). Within the brains of MS patients, both myelin-containing mDCs (CD209+) and pDCs (CD123+) have been observed in active demyelinating lesions, meninges and normal-appearing grey matter (Plumb et al., 2003; Cudrici et al., 2007; Lande et al., 2008). CD209+ DCs have also been observed in close association with lymphocytes in active MS lesions (Serafini et al., 2006). Both DC subtypes can also be found in the CSF of patients with neurological inflammatory disorders, whereby DC numbers are correlated with several parameters associated with CNS inflammation. Interestingly, cell surface expression levels of several molecules that drive adaptive immune responses (e.g. CD80, CD86 and CD40) were higher in mDCs in the CSF compared to blood-derived DCs (Pashenkov et al., 2001). pDCs express high levels of TLR9 and secrete type I interferons upon ligand binding (e.g. viral DNA). During MS relapses, increased numbers of pDCs have been reported in the CSF (without changes in the blood), consistent with observations that relapses may be driven by systemic viral infection (Longhini et al., 2011). In MS patients treated with natalizumab, a monoclonal antibody directed at the cell adhesion molecule α4-integrin (VLA4), the numbers of CD209+ DCs and CD4+ T cells were significantly decreased in cerebral perivascular spaces (del Pilar Martin et al., 2008). Patients treated with natalizumab also had decreased VLA4 expression on both pDCs and mDCs, with a resulting decrease in the ability of the DC to stimulate antigen-specific T cell responses (de Andres et al., 2012). Fingolimod (FTY720) is a recently approved oral treatment for MS and has a well-described mechanism of action that leads to sequestering of immune cells in secondary lymphoid tissues. In the context of DCs, FTY720 has been shown to reduce antigen presentation function, decrease pro-inflammatory cytokine production, increase IL10 and block in vivo trafficking (Lan et al., 2005; Muller et al., 2005; Durafourt et al., 2011). FTY720 can also promote an anergy-polarized phenotype of both immature and mature DCs (Zeng et al., 2012). Dimethyl fumarate (BG12) is in late clinical trials for the treatment of MS and has also been shown to inhibit DC maturation, decrease MHC class II and costimulatory molecule expression (CD80 and CD86), block IL6 and IL12 secretion and result in fewer activated Th1 and Th17 cells (Peng et al., 2012). In addition to MS, increased numbers of DCs have also been reported in the CSF of patients with bacterial meningitis and Lyme neuroborreliosis (Pashenkov et al., 2002). In the CNS of amyotrophic lateral sclerosis (ALS) patients, in addition to its animal models, DCs have also been observed in the ventral horn and corticospinal tracts, supporting a role for an immune response in patients with this neurodegenerative disease (Henkel et al., 2004). In Parkinson’s disease (PD), the discovery of anti-melanin antibodies in the sera of PD patients has prompted studies to investigate whether the innate immune system can be activated by this putative autoantigen. In vitro studies have shown that neuromelanin can be recognized by DCs and trigger their maturation, thus suggesting a rationale for a potential autoimmune mechanism driving the pathogenesis of PD (Koutsilieri et al., 2012). Both mDCs and pDCs have also been observed in the post-mortem brains of stroke patients (Yilmaz et al., 2010). In an experimental model of stroke, the anti-inflammatory actions of granulocyte colony-stimulating factor (G-CSF) are thought to be at least partly mediated by its effects on DCs. Administration of G-CSF led to a decrease in DC migration and maturation, in addition to reducing infarct size and improving clinical disability (Dietel et al., 2012). While many of these studies provide evidence that antigen presentation and immune system activation can occur within the CNS, animal studies have further demonstrated that DCs from the CSF can also migrate to cervical lymph nodes where they can activate adaptive immune responses, providing evidence that DCs may drive immune responses in both the CNS and periphery. It has been suggested that both CCR7 and CXCR4 (Cravens and Lipsky, 2002) chemokine receptors, which have been shown to be either elevated upon activation or expressed by mature DCs within the CSF (Kivisakk et al., 2004), may mediate migration of DCs into the lymph nodes. Of interest to CNS pathophysiology is the mechanism by which DCs cross the BBB and promote immune cell activation directly within the CNS. Migration of immune cells across the brain endothelial is complex, requiring cell tethering, rolling, attachment and extravasation. In addition, the expression of cell adhesion molecules, integrins and selectin molecules is important in initiating the attachment of immune cells to endothelial cells. Transmigration of immune cells across the BBB is also mediated by individual chemoattractant molecules called ‘chemokines’. CCL2, also known as monocyte chemotactic protein-1 (MCP1), is a chemokine that is important for recruiting monocytes, lymphocytes and DCs to sites of tissue injury (Seguin et al., 2003). In transgenic mouse models, it has been observed that CCL2 is essential for the attraction of mDCs into the CNS (Dogan et al., 2008). Conversely, overproduction of CCL2 in the CNS leads to accumulation of leukocytes across the endothelial basement membrane (Toft-Hansen et al., 2006). CCL2 expression in glial cells is increased in several CNS inflammatory states, including epilepsy (Foresti et al., 2009), Alzheimer’s disease (Sokolova et al., 2009), ischaemia (Yamagata et al., 2010) and EAE (Giraud et al., 2010). In addition to CCL2, other chemokines have also been shown to promote DC migration, including CCL20, CXCL10 and CCL3. Many of these chemokines are also expressed by CNS resident cells during inflammation (Serafini et al., 2000; Williams et al., 2009; Clarkson et al., 2012). Through the use of both transgenic animal studies and in vitro BBB modelling, studies have demonstrated the importance of these chemokines in DC recruitment into the CNS (Ambrosini et al., 2005; Zozulya et al., 2007; Wuest & Carr 2008). In vitro assays using a modified Boyden chamber model of human BBB endothelial cells have identified a subpopulation of monocytes that closely associate with endothelial cells and express DC markers (CD83 and CD209) (Ifergan et al., 2008). These cells have been termed endothelial-associated DCs (eDCs). The presence of these cells has been confirmed in situ within active MS lesions. The release of cytokines from brain endothelial cells, such as transforming growth factor beta (TGFβ) and granulocyte–macrophage colony-stimulating factor (GM-CSF), is thought to contribute to the differentiation of eDCs. eDCs are believed to be potent phagocytes; secrete IL12p70, TGFβ and IL6; and promote the proliferation of Th1 and Th17 CD4+ lymphocytes. Once DCs are in the CNS, there is evidence to support both a detrimental and beneficial role for them in inflammatory responses. As mentioned in this section, DCs can re-stimulate T cells and promote epitope spreading in the CNS. Adoptive transfer studies using the EAE model also demonstrate that MHC class II expression on DCs is sufficient to stimulate T cells and results in disease activity (Greter et al., 2005). DC production of IL12, a potent cytokine that stimulates Th1 responses, also suggests that some DC subtypes are capable of promoting adaptive immune responses in the CNS. pDCs in particular increase OX40L (CD252), which has been previously shown to promote Th2 responses (Kitajima et al., 2011). Studies have been performed in vivo whereby intracerebral injection of myelin oligodendrocyte glycoprotein (MOG)-loaded DCs prior to EAE induction significantly increased clinical disability and activation of infiltrating T cells (Zozulya et al., 2009b). In contrast, follow-up experiments using injections of DCs that were pre-exposed to TNFα induced DC tolerance, decreased IL17 and increased IL1 production, thus resulting in a delay or prevention of disease activity (Zozulya et al., 2009a). These findings may indicate that tolerogenic DCs may protect the CNS from immune-mediated injury and have important implications in further study of the roles of DCs in mediating CNS inflammatory injury. Microglia are specialized macrophages and glial cells within the CNS, and they have been suggested to originate from the yolk sac during development (Ginhoux et al., 2010). Early in development, microglia populate the CNS parenchyma where they have an extended lifespan with little turnover (Ginhoux et al., 2010, Rock et al., 2004). Fate-mapping studies have demonstrated that postnatal haematopoietic progenitors do not significantly influence homeostatic mechanisms specific to microglia in the adult brain. Microglia were first described by Pio del Rio-Hortega in 1932, and they have been reported to share many features with other tissue resident macrophages, with the exception of their resting ramified morphology (Rock et al., 2004). This unique morphology allows microglia to sense the local environment, communicate with neighbouring cells (e.g. neurons, oligodendrocytes, astrocytes) and quickly respond to infection and injury. Microglia express several cell surface and intracellular molecules, including CD14, CD11b, CD45, CD68 and EMR1 (F4/80 in rodents) (Prinz et al., 2011). Many of these molecules are also similarly expressed by monocyte and tissue macrophages. In mice lacking the PU.1 transcription factor, a critical transcription factor known to be important during myeloid and B cell development, microglia are notably absent in the neonatal CNS (Beers et al., 2006). Irradiation and bone marrow transplantation (BMT) studies have provided evidence that BMT-derived progenitors can efficiently migrate into the CNS (Eglitis & Mezey 1997, Priller et al., 2001). Animal studies using GFP knock-in CX3CR1 (fractalkine receptor) transgenic mice, whereby both peripheral macrophages and microglia are fluorescently labelled, have demonstrated that migration of GFP+ cells into the CNS occurs at E10.5, prior to the onset of embryonic haematopoiesis (Ginhoux et al., 2010).
Innate Immunity in the CNS – A Focus on the Myeloid Cell
Introduction to concepts of innate immunity
Cells of the innate immune system
Innate immune cell receptors
Cell Type
TLR
Ligand
Neuron
Astrocyte
Oligodendrocyte
Microglia
Brain Endothelium
TLR1
Triacylated lipoproteins
✓
✓
TLR2
Glycolipids, lipoproteings
✓
✓
✓
✓
TLR3
dsRNA
✓
✓
✓
✓
✓
TLR4
LPS
✓
✓
✓
TLR5
Flagellin
✓
✓
TLR6
Diacylated lipoproteins
✓
✓
TLR7
ssRNA
✓
✓
✓
TLR8
ssRNA
✓
✓
✓
TLR9
Unmethylated CpG-DNA
✓
✓
✓
Innate immune responses in the CNS
Roles of dendritic cells
Roles of microglia and macrophages
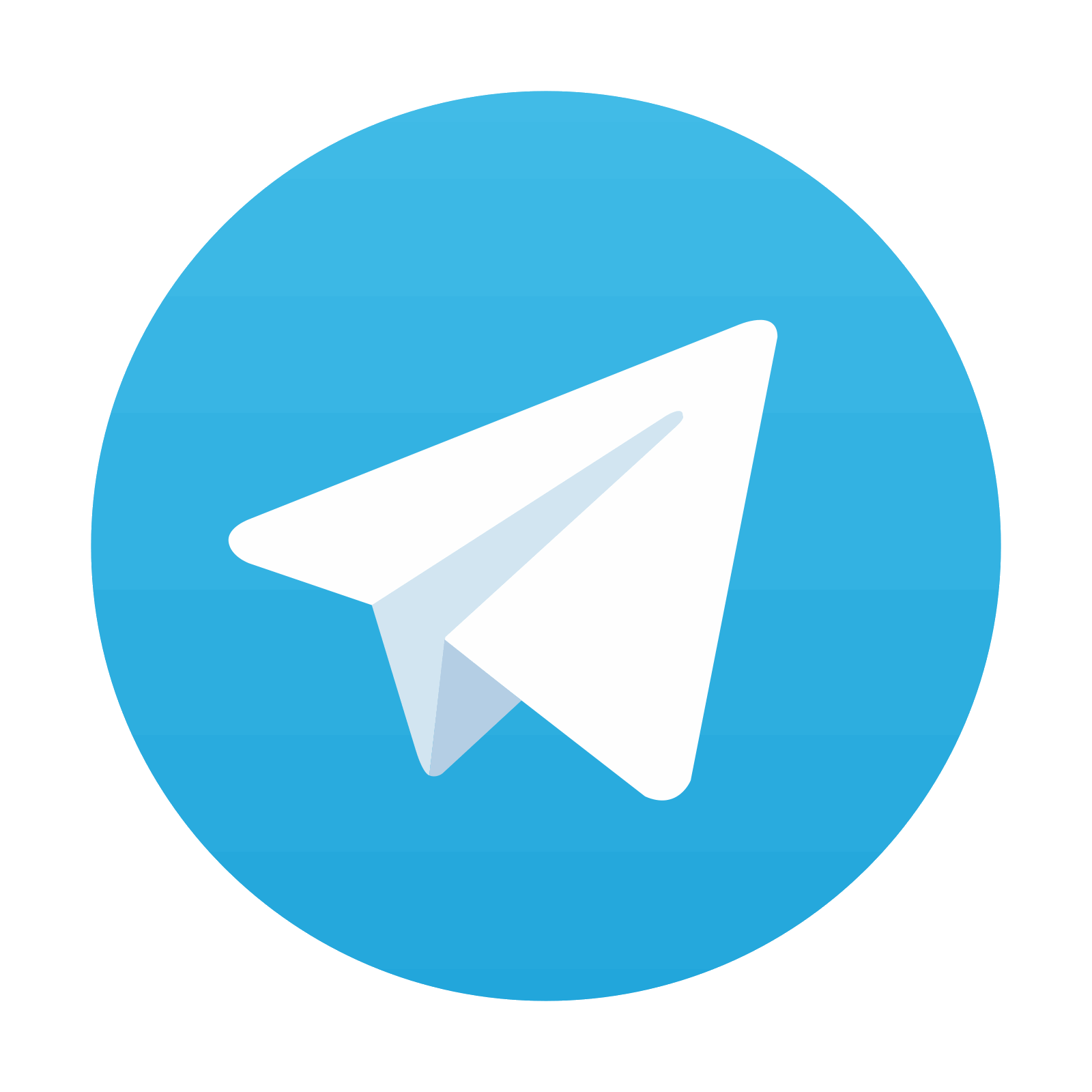
Stay updated, free articles. Join our Telegram channel
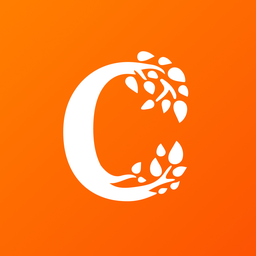
Full access? Get Clinical Tree
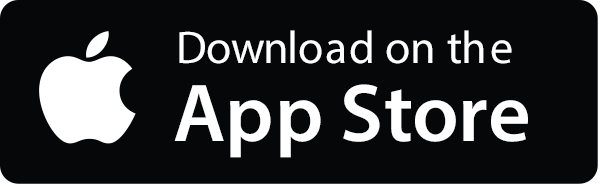
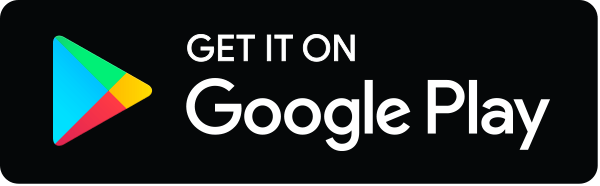