Stereotactic radiosurgery was conceptualized to treat functional diseases of the brain. The need for devices capable of molding the radiation dose to the nuances of intracranial lesions and yet preserve brain function became a challenge. Several devices capable of performing radiosurgery of high quality became commercially available, each with advantages and disadvantages. Speed of radiosurgery delivery for cost effectiveness and comfort for the patient are currently the main developments in the field. Nuances of these devices, procedural steps of radiosurgery, and the team approach of radiosurgery are discussed in this article.
Key points
Readers of this article will learn:
- •
The history of device development for radiosurgery.
- •
The technical nuances of each intracranial radiosurgery device.
- •
Step-by-step performance of a radiosurgery procedure.
- •
The need of a team approach in radiosurgery.
- •
The expansion of intracranial radiosurgery to other areas of the body.
Introduction
Stereotactic radiosurgery evolved based on two good ideas. First, treating a lesion in human tissues with external beam radiation, described by Kohl 18 years after the discovery of X-rays. The second hinged on the work of Horsley and Clarke, neurosurgeon and mathematician, respectively, who developed a tool to localize intracranial structures in three dimensions. This work resulted in a stereotactic atlas of the primate brain published in 1908. An atlas that combined the use of this development was the subject of Spiegel’s reported human stereotactic atlas in 1952.
The concept of applying focal X-rays as a therapeutic tool evolved using spiral converging beams, pendulum-directed beams, and finally rigid hemispheric distributed beams directed with stereotactic precision. It was Lars Leksell, a practicing functional neurosurgeon at Karolinska University in Stockholm, Sweden, who integrated stereotactic precision with the penetrating capability and the tissue effect of the photon beam. As widely described, Leksell attached an X-ray tube to his stereotactic arc centered frame and delivered radiosurgery to the first patient submitted to the technique, targeting the trigeminal ganglion for treatment of trigeminal neuralgia. The term “radiosurgery” was coined.
Radiosurgery evolved during the last half of the twentieth century linked to the explosion of imaging techniques. Because it was dependent on ventriculography, cysternography, and angiography, the applications of radiosurgery were largely limited to pathologies visualized by these techniques. Functional applications were based on principles of functional neurosurgery localization, for example using the anterior commissure and posterior commissure seen by ventriculography to guide targeting. Meckel cave contrast material injection and cysternography provided visualization of targets, such as the trigeminal ganglion in the Meckel’s cave and the acoustic neuroma’s prominence in the cerebellopontine angle, previously not seen in plain skull radiographs. Angiography provided the visualization of arteriovenous malformations (AVMs), making them the classic application of radiosurgery starting in 1972. The buildup of radiosurgery applications with the introduction of structural diseases, such as acoustic neuromas and AVMs, increased the demand for affordable radiosurgery throughout the world. During the early 1980s there were less than 10 radiosurgery devices serving the world’s population: three Gamma Knives and a few proton facilities.
Modern neurosurgery develops toward minimally invasive procedures, therefore radiosurgery has gained space. The multidisciplinary nature of the procedure involving the neurosurgeon, radiation oncologist, and medical physicist aims to minimize the risks and to improve the treatment success rate. This has been met with great acceptance by patients and payers alike. Radiosurgery has an important therapeutic role in the management of brain tumors, AVMs, and trigeminal neuralgia, and continues to expand its applications, including selected functional disorders of the brain, such as epilepsy.
The success of intracranial radiosurgery has also spread to the spine and other areas of the body, revolutionizing the practice of radiation oncology. The same impact of radiosurgery in general neurosurgery is being repeated in other surgical specialties, such as thoracic surgery. The clinical importance of radiosurgery expedited the development of new technologies capable of increased speed, comfort, and effects of radiosurgery for its diverse applications.
Today there are four major photon energy radiosurgery devices competing in the market based on advantages and disadvantages of respective intended specific applications and strategies of planning the treatment ( Table 1 ). Regardless of the approach, the fundamental concepts of radiosurgery include high doses of radiation, minimal doses in surrounding structures, stereotactic localization, use of computerized dosimetry planning, and a highly accurate radiation delivery system.
Modality | Indications | Key Features | Limitations |
---|---|---|---|
Circular collimator (single) | Small round targets, functional radiosurgery applications | Fast delivery, usually homogeneous (if diameter ≥10 mm) | Limited to small and round targets, rapid planning |
Multiple isocenters | Small-to-medium irregularly shaped target | Conformal, inhomogeneous | Slow delivery, inhomogeneous, time-consuming planning |
Dynamic-shaped beam | Small-to-medium irregularly shaped target | Conformal, homogeneous, fast radiation delivery | Loose conformality with large targets because of beam overlap |
Static-shaped beam | Large irregularly shaped target | Conformal, homogeneous, fast radiation delivery | More dose through the path of the beam, usually time-consuming planning |
Pencil beam painting | Irregularly shaped target | Conformal, homogeneous | Slow radiation delivery |
Intensity modulation | Large irregularly shaped target | Conformal, tighter dose distribution, better sparing of organ at risk | Slow radiation delivery, usually inhomogeneous, strict delivery quality control |
Introduction
Stereotactic radiosurgery evolved based on two good ideas. First, treating a lesion in human tissues with external beam radiation, described by Kohl 18 years after the discovery of X-rays. The second hinged on the work of Horsley and Clarke, neurosurgeon and mathematician, respectively, who developed a tool to localize intracranial structures in three dimensions. This work resulted in a stereotactic atlas of the primate brain published in 1908. An atlas that combined the use of this development was the subject of Spiegel’s reported human stereotactic atlas in 1952.
The concept of applying focal X-rays as a therapeutic tool evolved using spiral converging beams, pendulum-directed beams, and finally rigid hemispheric distributed beams directed with stereotactic precision. It was Lars Leksell, a practicing functional neurosurgeon at Karolinska University in Stockholm, Sweden, who integrated stereotactic precision with the penetrating capability and the tissue effect of the photon beam. As widely described, Leksell attached an X-ray tube to his stereotactic arc centered frame and delivered radiosurgery to the first patient submitted to the technique, targeting the trigeminal ganglion for treatment of trigeminal neuralgia. The term “radiosurgery” was coined.
Radiosurgery evolved during the last half of the twentieth century linked to the explosion of imaging techniques. Because it was dependent on ventriculography, cysternography, and angiography, the applications of radiosurgery were largely limited to pathologies visualized by these techniques. Functional applications were based on principles of functional neurosurgery localization, for example using the anterior commissure and posterior commissure seen by ventriculography to guide targeting. Meckel cave contrast material injection and cysternography provided visualization of targets, such as the trigeminal ganglion in the Meckel’s cave and the acoustic neuroma’s prominence in the cerebellopontine angle, previously not seen in plain skull radiographs. Angiography provided the visualization of arteriovenous malformations (AVMs), making them the classic application of radiosurgery starting in 1972. The buildup of radiosurgery applications with the introduction of structural diseases, such as acoustic neuromas and AVMs, increased the demand for affordable radiosurgery throughout the world. During the early 1980s there were less than 10 radiosurgery devices serving the world’s population: three Gamma Knives and a few proton facilities.
Modern neurosurgery develops toward minimally invasive procedures, therefore radiosurgery has gained space. The multidisciplinary nature of the procedure involving the neurosurgeon, radiation oncologist, and medical physicist aims to minimize the risks and to improve the treatment success rate. This has been met with great acceptance by patients and payers alike. Radiosurgery has an important therapeutic role in the management of brain tumors, AVMs, and trigeminal neuralgia, and continues to expand its applications, including selected functional disorders of the brain, such as epilepsy.
The success of intracranial radiosurgery has also spread to the spine and other areas of the body, revolutionizing the practice of radiation oncology. The same impact of radiosurgery in general neurosurgery is being repeated in other surgical specialties, such as thoracic surgery. The clinical importance of radiosurgery expedited the development of new technologies capable of increased speed, comfort, and effects of radiosurgery for its diverse applications.
Today there are four major photon energy radiosurgery devices competing in the market based on advantages and disadvantages of respective intended specific applications and strategies of planning the treatment ( Table 1 ). Regardless of the approach, the fundamental concepts of radiosurgery include high doses of radiation, minimal doses in surrounding structures, stereotactic localization, use of computerized dosimetry planning, and a highly accurate radiation delivery system.
Modality | Indications | Key Features | Limitations |
---|---|---|---|
Circular collimator (single) | Small round targets, functional radiosurgery applications | Fast delivery, usually homogeneous (if diameter ≥10 mm) | Limited to small and round targets, rapid planning |
Multiple isocenters | Small-to-medium irregularly shaped target | Conformal, inhomogeneous | Slow delivery, inhomogeneous, time-consuming planning |
Dynamic-shaped beam | Small-to-medium irregularly shaped target | Conformal, homogeneous, fast radiation delivery | Loose conformality with large targets because of beam overlap |
Static-shaped beam | Large irregularly shaped target | Conformal, homogeneous, fast radiation delivery | More dose through the path of the beam, usually time-consuming planning |
Pencil beam painting | Irregularly shaped target | Conformal, homogeneous | Slow radiation delivery |
Intensity modulation | Large irregularly shaped target | Conformal, tighter dose distribution, better sparing of organ at risk | Slow radiation delivery, usually inhomogeneous, strict delivery quality control |
Basic concepts
Ionizing Radiation
Ionizing radiation for radiosurgery is any radiant entity that has enough energy to remove an electron from an atom, thus creating ions, which interact with the living tissue in the target generating a biologic response. Gamma rays are ionizing radiation originating from an excited nucleus of cobalt 60. This nucleus continuously gets rid of excess energy by emitting electromagnetic radiation, known as gamma ray. X-rays, however, are generated when an energized electron hits a heavy weight metal and looses energy in the form of a photon. This electromagnetic species has the same biologic effect on the targeted living tissue as a gamma ray. These photon beans generate free radicals that interact with molecules of DNA causing cell death, cell inability to divide, or modification of cell function. Radiosurgery takes advantage of the linear propagation of electromagnetic energy and its ability to interact with living matter to provoke the intended therapeutic reaction in the target tissue.
Another important factor of ionizing radiation for radiosurgery is the particulate energy. Because of the unique capability to stop its propagation at the point of maximal energy delivery in the tissue, a phenomenon called Bragg peak, it is very attractive for radiosurgery. It spares the tissue beyond the target, contrary to photons that irradiate beyond the target site. Particulate energies are produced in large cyclotrons where electrons are spanned out of the outer atomic layer and the nuclei are directed to the intended target by powerful magnets. In the case of protons, the hydrogen ion is the one spanned in the cyclotron. Historically, protons and alpha particles prominent of spanned helium beams established radiosurgery for pituitary tumors and had a major impact in the treatment of AVMs. Because of its at least 30 times order of magnitude price compared with photon-based techniques, the particulate energy was used in only a few academic centers worldwide. Modern particle accelerators dedicated to medical purpose are starting to become available. However, the price is still at least of 10 orders of magnitude of the current available dedicated radiosurgery system on the market.
Radiosurgery Procedure
Understanding all of the steps of the radiosurgery procedure is essential to optimize results and reduce risks. Regardless of the method of delivery, it is important to follow all the safety steps of the procedure, because there is no equipment that is human error proof. Moreover, radiosurgery is prone to repetitive error. Because the effects of the treatment are not immediately seen, a large number of patients can be treated based on a single human error. Radiosurgery should not be performed without a very well trained team made up of radiation technologists, medical physicists, radiation oncologists, and neurosurgeons.
The first step is a daily quality assurance routine to check basic aspects of the delivery system and software, and the precision of the delivery device. This should be followed by correct application of the stereotactic guiding device, either frameless or with a frame. A routine protocol of treatment delivery followed harmonically by all team members is essential.
The preparation for the procedure is based on acquisition of exquisite quality imaging dictated by the pathology. Integration of all imaging modalities, such as computed tomography (CT), CT angiography, magnetic resonance imaging, magnetic resonance angiography, conventional angiogram, and positron emission tomography, is desirable in modern radiosurgery systems. The treatment plan consists of a careful analysis of the image quality and the fusion of all images available to be registered in the same stereotactic space. The target volume is defined based on the composite of information of all images. The best dose distribution to affect the target and spare normal structures surrounding the disease is the goal of radiosurgery treatment, requiring the expertise of the physicist, the radiation oncologist, and the neurosurgeon. Each one brings to the radiosurgery plan the understanding of dose deposition, dose effect, and anatomic pitfalls known by each specialty. All specialists evaluate the isodose distribution, the dose volume histogram, the conformity index, and the feasibility of the radiosurgery system to delivery reliably and in an acceptable time frame the treatment plan.
Radiosurgery Team
Radiosurgery is based on a multidisciplinary concept. The physicist brings knowledge of the device, the neurosurgeon of the anatomy, and the radiation oncologist of the interaction of ionizing radiation with living tissue. This concept generated a novel specialty overlap but none of the domains is able to independently perform the procedure within safe and optimized standards. In the operating room the neurosurgeon needs the anesthesiologist, the specialized nurse, the technicians, the electrophysiologist, and so on. Similarly, in radiosurgery the multiple professionals are necessary. This concept has been met with resistance in certain institutions, mainly related to the ego driven in each specialty and the economic interests of each specialist. This has led to major complications, harm to patients, and degradation of the method. Because the development of a field hinges on a well-established base of knowledge, radiosurgery only progresses when the basics of physics, radiobiology, anatomy, and clinical skills are well established. This occurs only with the participation of professionals working and dedicated to each of these fields of knowledge. Although economic pressure leads certain institutions to abolish or ostracize one of the components of the team, the consequences are lack of progress, increased risks for the patients, and poor results. The harmonic work of the radiosurgery team has provided for a minimally invasive approach, better quality of life for patients, and novel therapies for yet untreatable diseases. Radiosurgery has taken a position of being responsible for 15% to 30% of the neurosurgery cases in major departments of neurosurgery.
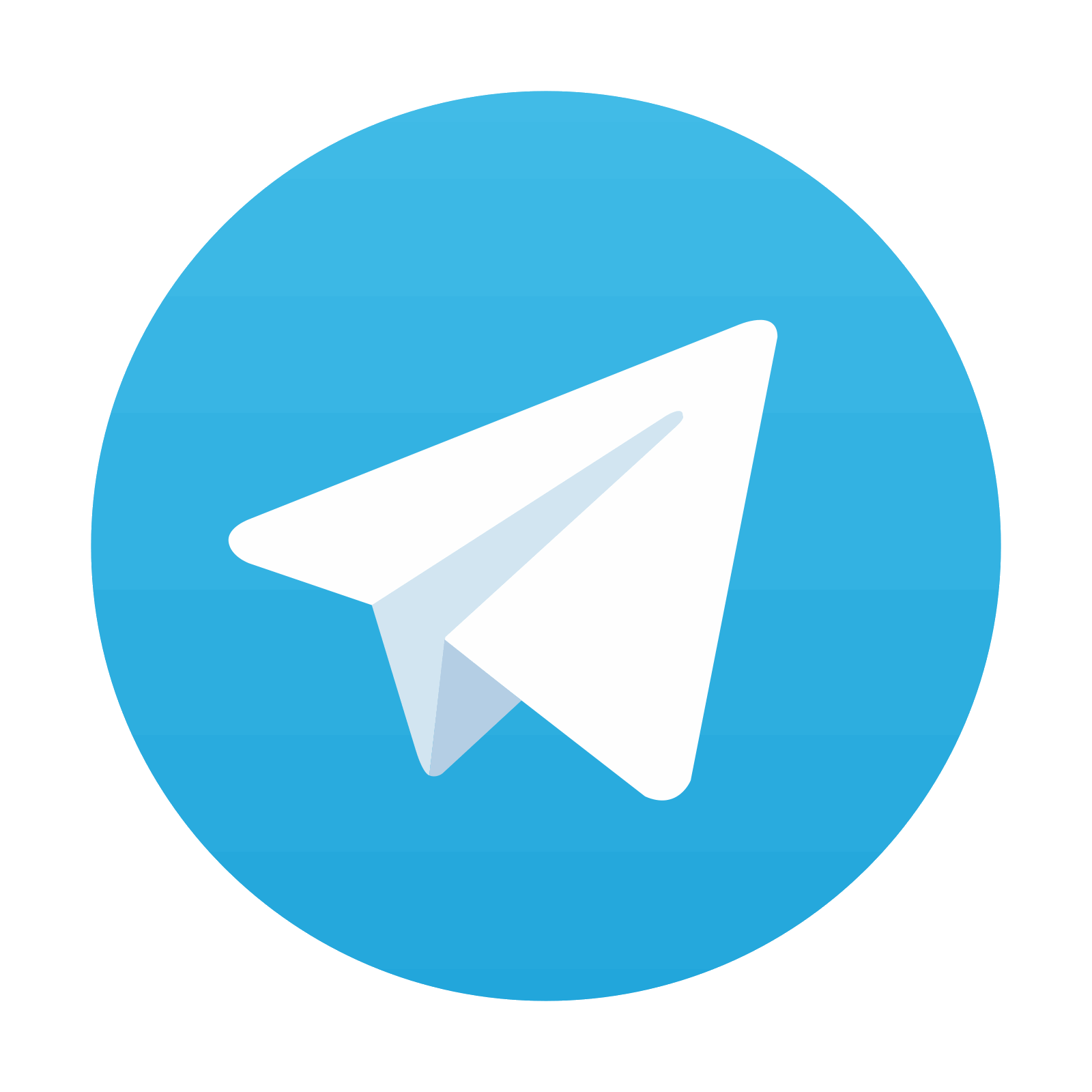
Stay updated, free articles. Join our Telegram channel
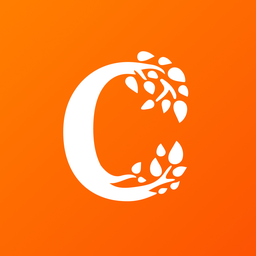
Full access? Get Clinical Tree
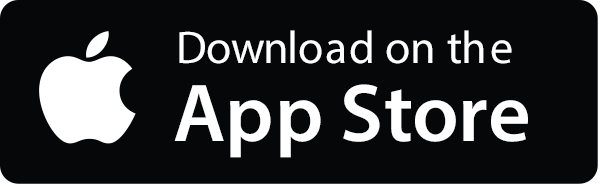
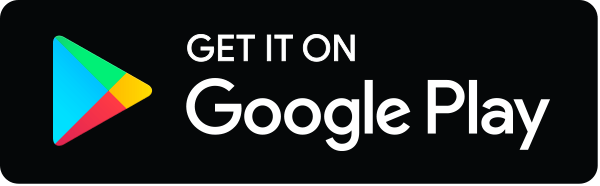