Multimodal functional navigation enables removing a tumor close to eloquent brain areas with low postoperative deficits, whereas additional intraoperative imaging ensures that the maximum extent of the resection can be achieved and updates the image data compensating for the effects of brain shift. Intraoperative imaging beyond standard anatomic imaging, that is, intraoperative functional magnetic resonance imaging (fMRI) and especially intraoperative diffusion tensor imaging (DTI), add further safety for complex tumor resections. This article discusses the acquisition of intraoperative fMRI, DTI, and imaging.
Functional navigation
The integration of functional data from functional MRI (fMRI) and magnetoencephalography (MEG) identifying eloquent cortical brain areas into standard 3-dimensional (D) anatomic datasets is known as functional navigation. Eloquent functional structures, such as the motor strip or language-related areas can be identified during surgery by visualizing their position using the microscope-based heads-up display technology. Functional navigation allows resection of tumors close to eloquent brain areas with low postoperative deficits, whereas intraoperative imaging ensures that the maximum extent of a resection can be achieved.
Especially in surgery of high-grade tumors, it is of no benefit to maximize the extent of a resection to potentially increase the survival time by only some weeks when risking permanent neurologic deficits right after surgery. It is absolutely mandatory to combine the goal of maximum resection with the goal of preservation of function. Intraoperative imaging helps to maximize the extent of resection, and in combination with functional multimodal navigation it helps to minimize postoperative neurologic deficits. With the advances in surgical techniques and perioperative technology, it is now possible to maximally resect malignant intrinsic glial neoplasms, even close to functionally critical areas, without increased morbidity. Studies have demonstrated a survival advantage in these lesions with a resection extent of 98% or greater, particularly in younger patients with good Karnofsky scores.
To achieve this advantage, functional navigation, that is, integrating functional data into anatomic navigational datasets, is an important add-on to intraoperative MRI because it prevents too extensive resections, which would otherwise result in new neurologic deficits. Meanwhile, data from MEG and fMRI are routinely integrated in functional navigation, allowing identification of eloquent brain areas, such as the motor area and speech-related areas.
In a retrospective study, the author’s group investigated how the decision to resect a glioma was influenced by MEG. Altogether, 191 patients were examined, 119 of them harbored supratentorial gliomas. About every forth patient (26.8%) yielded a severe possible danger of postoperative neurologic morbidity according to MEG and thus was not considered to be a good candidate for surgery. This result corresponds to published data in which 12 of 40 investigated patients (30%) with tumors and vascular malformations underwent nonsurgical therapy based on the MEG results. When functional data were used in combination with frameless stereotactic devices the postoperative morbidity was as low as 2.3%. However, the overall morbidity was 6.8%. These data reflect the beneficial effects of functional navigation in comparison to data of other studies with morbidity rates varying from 6% to 31.7%. These figures can also be interpreted as a result of a more careful patient selection based on preoperative brain mapping. Preoperative identification of eloquent brain areas has an effect in the risk evaluation in glioma surgery, and functional navigation reduces the risk for postoperative neurologic deficits. Besides identification of the motor strip, the localization of language areas has a great clinical effect.
The concept of functional navigation was expanded by the integration of further data leading to the so-called multimodal navigation. To prevent postoperative neurologic deficits, it is also mandatory to preserve major white matter tracts, such as the pyramidal tract for the motor system, that are connected to eloquent cortical brain areas, which are localized by fMRI and MEG.
As a first attempt to integrate information about major white matter tracts into a multimodal navigation setup, diffusion data with limited directional information were used for a rough estimation of the course of the pyramidal tract and applied for intraoperative guidance during brain tumor resection. These methods often required time-consuming preoperative manual data processing, such as the segmentation of the assumed major white matter tract in single slices and subsequent image registration. The reliability of the reconstructed white matter tract depended mainly on the experience of the individual person processing the data.
Diffusion tensor imaging (DTI) is based on measuring multiple diffusion-weighted images in different gradient directions to resolve the orientation of the white matter tracts. DTI can resolve the dominant fiber orientation in each voxel element. The direction of greatest diffusion measured by DTI parallels the dominant orientation of the tissue structure in each voxel, representing the mean longitudinal direction of axons in the major white matter tracts. DTI provides information about the normal course, the displacement, or interruption of white matter tracts around a tumor, and the widening of fiber bundles due to edema or tumor infiltration can be detected.
To integrate DTI data into navigational datasets, the first step was to register fractional anisotropy (FA) maps with the standard anatomic 3D images. In contrast to using diffusion-weighted images for the delineation of major white matter tracts, the manual segmentation in color-coded FA maps was more reliable and less user dependent. However, still it was a time-consuming process, which allowed, especially close to a lesion, only a rough and quite unreliable estimation of the course of the pyramidal tract.
Fiber tracking is probably the most clinically appealing and understandable technique for representing major white matter tracts. Various tracking algorithms that compare local tensor field orientations measured by DTI from voxel to voxel have been developed, allowing a noninvasive tracing of large fiber tract bundles in the human brain ( Fig. 1 ).

Initially, most approaches did not satisfy the needs of neurosurgical planning. For clinical intraoperative use, the actual border of a major white matter tract is of interest. A line representation such as the visualization in standard fiber tracking lacks the ability to provide a border, so the user has to interpret the visualization as a model for the tract. The generation of hulls is a possibility to overcome this drawback. A surface wrapping a particular subset of previously computed streamlines represents a certain fiber tract bundle. This representation results in an intuitive visualization with flexible tubes, which is closely related to the expected appearance of a major white matter bundle or a complete 3D object representing the major white matter tract of interest. The combination with volume rendering of anatomic magnetic resonance (MR) data provides a good spatial orientation. Alternatively to this wrapping approach, volume-growing techniques can also generate 3D objects representing major white matter tracts.
Integration of tractography data into a stereotactic coordinate system was the next major step in the development of major white matter tract navigation. Most of these applications and approaches, however, were stand-alone applications developed for individual clinical sites. A broad application for routine clinical use was not possible with the various prototype applications, and the missing standardization did not allow comparing the different approaches.
The implementation of a fiber tracking algorithm into a standard navigation system, allowing routine usage and broad availability, solved these restrictions. Registration with standard anatomic image data greatly facilitated the generation and selection of the fibers of interest as well as eased the delineation of the relationship of the tracked fibers to certain anatomic structures because the seed regions for the tracking algorithms could be defined in high-resolution 3D anatomic images. The implemented approach allowed a straightforward definition of volumes of interest for selecting the fiber tracts of interest. Only 2 parameters, the FA threshold and the minimum length of the fibers that will be computed, have to be selected by the user. The total generation of the fiber tracts, including image transfer, registration of the diffusion data with the standard anatomic image data, tensor calculation, fiber tracking, and the final generation of a 3D object, needs less than 10 minutes, depending to some extent on the strategy by which the different seed volumes of interest during initiation of the tracking algorithm are selected.
The intraoperative visualization of the course of the pyramidal tract by microscope-based navigation during the resection of supratentorial gliomas has resulted in reduced neurologic deficits, which may serve as a proof of the concept per se. This concept is also supported by studies comparing preoperative and postoperative reconstructions of major white matter structures in the brain stem well correlating to clinical deficits. Visual field deficits in temporal lobe surgery for pharmacoresistant epilepsy provide an ideal model to analyze the clinical validity of changes in fiber tracking by correlating the extent of visual field defects with the changes in preoperative and intraoperative DTI-based reconstruction of the optic radiation. The significant correlation between postoperative visual field deficits and the extent of alterations of the optic radiation also proved that reconstruction of major white matter tracts can be reliably used in a clinical setting.
All these data clearly support the concept of functional navigation, that is, adding functional information to 3D anatomic datasets to reduce postoperative morbidity when operating on lesions close to eloquent brain structures. Of course, intraoperative knowledge of the exact position of the pyramidal tract does not prevent neurologic deficits per se; intraoperative events, such as the necessity to coagulate small vessels close to the pyramidal tract, may result in an injury of the pyramidal tract leading to neurologic deficits. The closest distance to which a reconstructed major white matter tract can be approached is not yet clearly defined. Analyzing the DTI-based navigation data with regard to the distance between tumor and pyramidal tract revealed that a distance of 5 mm seems to be a critical distance, which should be taken into account as safety margin. This value corresponds well to an identical critical distance of about 5 mm when approaching functionally eloquent cortical brain areas delineated by fMRI or MEG data.
Additional hulls around the reconstructed 3D objects representing major white matter tracts make visualization of these safety margins possible. Ideally, these encompassing hulls would vary in thickness with respect to the quality and reliability of the reconstructed fiber bundle. In case of noisy unreliable data a thick hull would be added, whereas in highly reliable data the hull would be thinner. The technical and clinical definition of the extent of these safety margins has still to be established.
The major question is whether the tracking results actually reflect reality. First attempts correlating the DTI findings to intraoperative electrophysiological measurements showed quite some discrepancies, which are probably mainly attributable to a distinct shifting of major white matter tracts during a neurosurgical procedure, which could be demonstrated by comparing preoperative and intraoperative fiber tracking, acquired by high-field MRI applied during surgery.
Intraoperative imaging
Navigation systems have a decreasing accuracy during the course of a surgical procedure because these systems are normally based on preoperative data only. This decreasing accuracy is because of brain shift, that is, the intraoperative deformation of the brain, which is caused by tumor removal, brain swelling, the use of brain retractors, and cerebrospinal fluid drainage. Intraoperative imaging offers a possibility to compensate for the effects of brain shift. It depicts a virtual reproduction of the actual intraoperative physical reality, on how the brain is deformed and on the extent of tumor removal. The ability to objectively determine the extent of tumor removal during surgery is of great value. If a resection is incomplete, tumor residues that were initially missed can be further removed during the same operation. Intraoperative imaging allows an objective evaluation of the actual intraoperative situation, thus serving as quality control during surgery.
Intraoperative high-field MRI is the most sophisticated possibility for intraoperative imaging compared with the alternatives such as intraoperative computed tomography and intraoperative ultrasonography. Combining high-field MRI and microscope-based navigation enables an intraoperative possibility to compensate for the effects of brain shift by intraoperatively updating the image information. For the update, the intraoperative images have to be registered either by a calibrated registration matrix that is attached to the upper part of the imaging coil and tracked by the navigation system or by a direct registration of preoperative and intraoperative images, assuming that there is no positional shift, that is, that the initial patient registration was correct, so that the coordinate system of the initial patient registration can also be applied for the intraoperative images. Updated navigation with intraoperative image data allows a reliable identification of tumor remnants. Microscope-based heads-up displays with the direct visualization of the segmented tumor remnant in the surgical field play a crucial role in the precise localization and orientation in the resection cavity.
However, these updates typically are updates of anatomic data, so that the initially integrated preoperative functional data are lost. On the other hand, intraoperative high-field MRI not only allows for standard anatomic imaging such as T1- and T2-weighted sequences but also makes intraoperative fMRI and, more important clinically, intraoperative DTI feasible. Intraoperative MR spectroscopy (MRS) still has its limitations because of the brain-air interface. So there remains some distinct challenges for updating also the MRS information intraoperatively.
Intraoperative imaging
Navigation systems have a decreasing accuracy during the course of a surgical procedure because these systems are normally based on preoperative data only. This decreasing accuracy is because of brain shift, that is, the intraoperative deformation of the brain, which is caused by tumor removal, brain swelling, the use of brain retractors, and cerebrospinal fluid drainage. Intraoperative imaging offers a possibility to compensate for the effects of brain shift. It depicts a virtual reproduction of the actual intraoperative physical reality, on how the brain is deformed and on the extent of tumor removal. The ability to objectively determine the extent of tumor removal during surgery is of great value. If a resection is incomplete, tumor residues that were initially missed can be further removed during the same operation. Intraoperative imaging allows an objective evaluation of the actual intraoperative situation, thus serving as quality control during surgery.
Intraoperative high-field MRI is the most sophisticated possibility for intraoperative imaging compared with the alternatives such as intraoperative computed tomography and intraoperative ultrasonography. Combining high-field MRI and microscope-based navigation enables an intraoperative possibility to compensate for the effects of brain shift by intraoperatively updating the image information. For the update, the intraoperative images have to be registered either by a calibrated registration matrix that is attached to the upper part of the imaging coil and tracked by the navigation system or by a direct registration of preoperative and intraoperative images, assuming that there is no positional shift, that is, that the initial patient registration was correct, so that the coordinate system of the initial patient registration can also be applied for the intraoperative images. Updated navigation with intraoperative image data allows a reliable identification of tumor remnants. Microscope-based heads-up displays with the direct visualization of the segmented tumor remnant in the surgical field play a crucial role in the precise localization and orientation in the resection cavity.
However, these updates typically are updates of anatomic data, so that the initially integrated preoperative functional data are lost. On the other hand, intraoperative high-field MRI not only allows for standard anatomic imaging such as T1- and T2-weighted sequences but also makes intraoperative fMRI and, more important clinically, intraoperative DTI feasible. Intraoperative MR spectroscopy (MRS) still has its limitations because of the brain-air interface. So there remains some distinct challenges for updating also the MRS information intraoperatively.
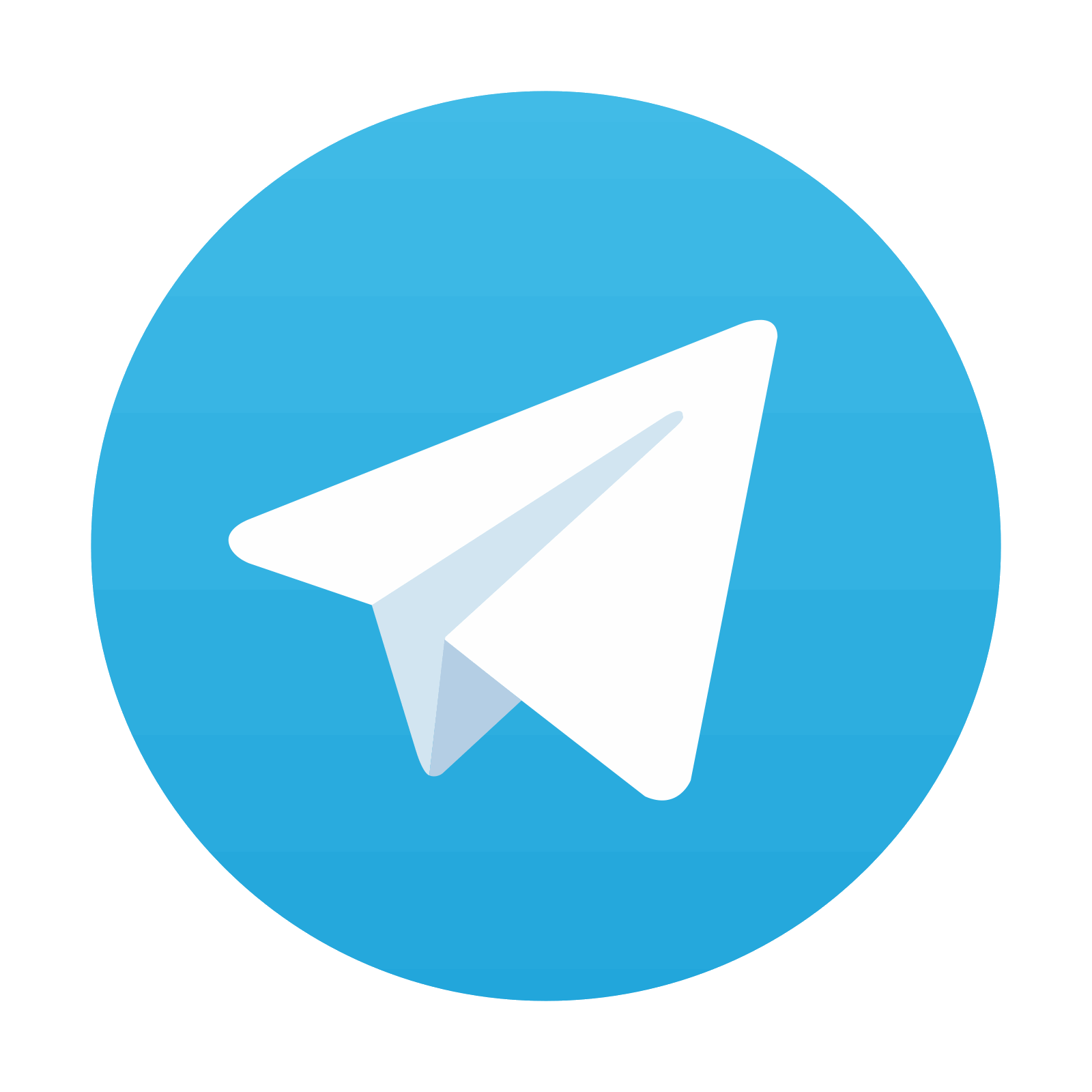
Stay updated, free articles. Join our Telegram channel
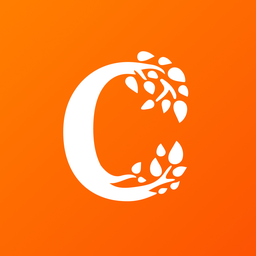
Full access? Get Clinical Tree
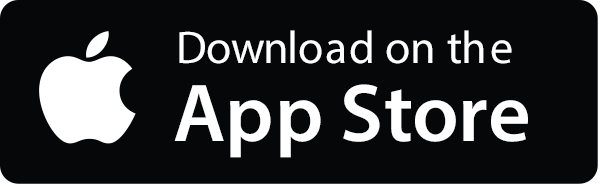
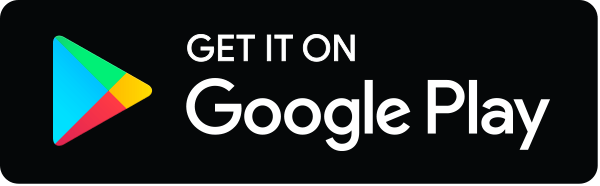