Introduction
Intraoperative adjuncts of various kinds hold a very prominent and revered place in the modern neurosurgery armamentarium. In addition to routine frameless neuronavigation which is almost ubiquitously used, intraoperative magnetic resonance imaging (MRI) is used to optimize brain tumor resections , intraoperative angiography is used for the resection of cerebral vascular malformations , intraoperative ultrasound is used to place shunt catheters in the ventricles of the brain or into various cysts , intraoperative electrophysiological monitoring has become a mainstay for the surgical removal of the spinal cord and brainstem lesions , while robotic-assistance now dominates the implantation of stereo-electroencephalography (sEEG) electrodes . Commonly used techniques of microsurgery and neuroendoscopy, which we now take for granted are based entirely on taking advantage of intraoperative adjuncts, namely microscopic and endoscopic visualization, respectively . Most, if not all, of these surgical adjuncts have been widely accepted as beneficial or even obligatory in each of their various niches. However, the benefits of the use of specific intraoperative adjuncts for focal epilepsy resective surgery have not been as clearcut . The root of this difference is that epileptogenic lesions are often less visible on even the most advanced neuroimaging and even under the operative microscope; the epilepsy surgeon is often operating on a hypothesized epileptogenic zone (EZ) , based on an extensive multimodal presurgical evaluation , and using the intraoperative adjunct as a method to fine-tune or “fill in the blanks” in the real-time setting. With the well-known limitations of each method in mind (discussed below), it requires a leap of faith to walk the thin line between removing abnormal epileptogenic tissue while not encroaching into the normal brain with the use of these imperfect methods. Usually, the outcome of such an endeavor can only be as good as the hypothesis going in, but optimization of some of the existing (and future) intraoperative adjuncts for epilepsy surgery holds promise to potentially improve on past shortcomings. New exciting technologies have been introduced , but “new” and “exciting” do not always translate into efficacious, beneficial and worthwhile; “all that glitters is not gold.” No matter how attractive a single technology may be, a “one size fits all” approach is not always best for patients. Rather, it is key to take a more tailored approach using different intraoperative adjuncts, or combinations thereof, for the particular cases that most suit these technologies.
The Challenge of Surgically Removing Epileptogenic Pathologies Completely When They Cannot be Completely Seen
As alluded to, the most important element of the surgical management of focal epilepsy is the presurgical evaluation itself . The modern multimodal neuroimaging and neurophysiological evaluation involves EEG, MRI, fluorodeoxyglucose (FDG)-positron emission tomography (PET), single-photon emission computed tomography (SPECT), EEG-functional MRI (EEG-fMRI), magnetoencephalogram (MEG), as well as intracranial recording . This determines whether a patient is a surgical candidate and provides the “best hypothesis” regarding the localization of the EZ and its borders. However, no matter how good this presurgical hypothesis is, none of the aforementioned presurgical evaluation tests can accurately delineate these EZ borders. Even in the case of MRI-visible malformations of cortical development (MCD), such as focal cortical dysplasia (FCD) Type IIb, it is now well-known that such lesions extend beyond what can be seen with even 3 T MRI (the utility of 7 T MRI in this regard remains to be determined) . The intracranial recording is considered the gold standard for EZ determination and based on the sEEG work of Talairach and Bancaud more than a half-century ago , areas of early spread (areas of primary organization of epileptic activity) have also been used to predetermine the borders of resection . The idea is that epileptogenic tissue ends somewhere between the seizure onset zone (SOZ) and the areas of early spread (i.e., the EZ includes the SOZ and the areas of early spread); in theory, this would allow for the surgical removal of all the SOZ (and all the EZ) if the resection was at least brought to areas of early spread . However, due to the limitation of spatial coverage of sEEG there are many areas around the SOZ which do not have electrodes. Thus, all areas of early spread cannot be determined in all 3D directions and depths. Hence, no current presurgical evaluation tool can fully delineate the borders of the EZ. As such, there has always been a desire to have some real-time intraoperative method to safely optimize the extent of resection. The main attempts at intraoperative technological assistance for epilepsy surgery have come in three forms which are not surprising: electrophysiology, neuroimaging, and real-time indirect histopathology assessment.
The Past: Intraoperative Electrophysiology
Naturally, the first intraoperative adjunct used in an attempt to optimize the extent of resection was electrocorticography (ECoG). Pioneered by Penfield and Jasper at the Montreal Neurological Hospital in the 1930s , electrodes were placed on the exposed surface of the brain ( Fig. 5.1 ) during surgery for mapping eloquent cortex during awake craniotomy, but also to record interictal activity to help determine how much of the cortex should be removed during epilepsy surgery. While initially adopted and widely popularized by epilepsy surgeons throughout the world, the trust in this method has waned in recent years . Not only has ECoG never been formally shown to improve epilepsy surgery outcomes in a head-to-head comparison with non-ECoG surgeries, but by the turn of the century, many criticisms had piled up against it. First, ECoG usually only records interictal epileptiform discharges (IEDs), not seizures. These IEDs may not only signify the parts of the cortex that need to be removed but also innocent bystander irritative zones, which also raises the concern that such innocent IEDs could be recklessly followed into normal, even eloquent brain regions. Indeed, this so-called “chasing of spikes” could lead to unnecessary neurological deficits, especially if some of these spikes are artifacts of cortex irritation from the surgery itself and/or the electrodes touching the surface of the brain . Some spikes are believed to be benign and not correlated to postsurgical seizure outcome, while others are thought to be protective, but this remains controversial . Furthermore, due to the time constraints of open epilepsy surgery, ECoG is typically only performed for short periods of time (approximately 20–30 minutes), during which informative or representative IEDs may not be recorded at all. In addition, various anesthesia agents can increase or decrease IEDs , thereby artificially altering the recording, and like routine scalp EEG, there can be interobserver disagreement in the interpretation of the recording.

Keeping in mind the limitations listed above, some cases are thought to be less amenable to ECoG and others more amenable to this intraoperative adjunct. Specifically in surgery for mesial temporal sclerosis (MTS), it has been shown that residual spikes on ECoG do not correlate with seizure outcome, and thus ECoG is recognized as not being helpful for such cases . On the other hand, due to the intrinsic epileptogenicity of FCD, with its characteristic electrographic signature (i.e., repetitive pacemaker-like IEDs), this pathology is thought to be somewhat more amenable to ECoG-based EZ delineation. Indeed, several studies and a recent meta-anaysis have reported the benefits of ECoG for FCD , but still the rate of postsurgical seizure freedom in such studies has hovered around 50%, which may again be due to the limitations of interpreting ECoG within the time constraints, signal-to-noise ratio constraints, and other artifactual constraints of open neurosurgery.
High-Frequency Oscillations
However, proponents of ECoG should not despair. Interest in ECoG has been rejuvenated over the past several years with the promise that high-frequency oscillations (HFOs), the much-celebrated electrophysiological biomarker of extra-operative intracranial recording, could also be utilized in the operating room . HFO frequency bands are defined as ripples (80–250 Hz) and fast ripples (250–500 Hz). Many studies using SEEG, subdural grids, and retrospectively analyzed ECoG, have assessed the association of HFOs with the EZ . In particular, fast ripples are highly specific markers of the EZ, and more specific than IEDs . In addition, these are believed to be produced only in abnormal histopathological parts of the brain and are less prone to artifactual generation during ECoG . Retrospective evaluation of ECoG recordings for the extent of resection of fast ripples has been shown to correlate with seizure freedom in several studies . However, a recent randomized controlled trial showed that HFO-guided tailoring of epilepsy surgery was not noninferior to spike-guided tailoring, except for in extratemporal lobe epilepsy surgeries . Therefore, the debate goes on but we certainly say that detection of fast ripples during ECoG is a very attractive and active area of investigation presently.
However, the real-time intraoperative recording, detection, analysis, and interpretation of HFOs have many challenges. First, the institute wanting to use intraoperative ECoG for real-time HFO analysis needs an established set-up of being able to record at least at 2000 Hz in the operating room (which not all portable EEG machines can do) and an experienced team to perform the HFO analysis . This requires an automatic detector that has already been tested retrospectively on intraoperative ECoG data to establish a consistent correlation between intraoperative ECoG HFO findings, their resection, postsurgical outcome and most importantly, safety . After this groundwork is completed, this endeavor would require a lot of preparation and good communication between the neurosurgery team, the epileptologist who will monitor the ECoG in the operating room, and the team performing the remote HFO analysis, for each case. Furthermore, it will take much effort to optimize the signal-to-noise ratio of the ECoG recording in the operating room, which sometimes means turning off surrounding machines and other equipment. The ECoG recording length during the surgery will have to be kept relatively short to compensate for other time delays of the pipeline, as once the ECoG data is recorded it needs to be transferred to the remote team doing the analysis. Then some time is needed for the interpretation of the results before these are relayed back to the epileptologist and neurosurgeon in the operating room. A 4×8 subdural grid of only 32 contacts is required, which is much less than that of a typical SEEG implantation (often >100 contacts). Therefore, the size of files for transfer and automatic detection is probably not the limiting factor, unlike the challenging operating room environment. To our knowledge, no center is routinely using intraoperative ECoG for HFOs analysis to make real-time decisions during surgery, but this is likely on the horizon. The rationale for this is sound and if some of these intraoperative environment hurdles can be overcome, this may prove to be one of the most promising intraoperative adjuncts for epilepsy surgery to date.
However, the use of electrophysiological biomarkers to delineate underlying histopathology is also not perfect. What if some underlying or adjacent histopathology is not active (i.e., not produced IEDs or even HFOs) at the time of the surgery? What if it is not active during the time of the presurgical evaluation but could become active in the future? A larger-scale analogy would be the multiple tubers of the tuberous sclerosis complex. We can remove the causative tuber during one surgery, but a year later, another tuber can become active leading to new seizures. It is possible that areas of FCD or even hippocampal sclerosis may be left behind during an ECoG-based surgery because these areas had no IEDs and no fast ripples at the time, but months or years later such areas start producing seizures. This partially explains the patients who initially become seizure-free after surgery, and do well for many years, only to have their seizures return 5 or 10 years later . To prevent this, we would need some intraoperative adjunct that could detect all the histopathology at the time of surgery. The luxury of many epileptogenic pathologies is that they are static over time; MCD do not grow or progress. The benign tumors may grow slowly but at least this can be mostly well-seen on MRI.
The Present: Neuronavigation and Intraoperative Neuroimaging
Pathologies That Can be Seen Well on Magnetic Resonance Imaging
It is well known that finding a lesion, such as MTS, a tumor, or a cavernous malformation on MRI is an excellent prognostic factor for a patient with focal epilepsy. Surgery for MTS provides more than 70% seizure freedom . If the lesion appears to be a benign brain tumor such as a ganglioglioma, DNET, or pilocystic astrocytoma, the prognosis is even better, with over 80% long-term postsurgical seizure freedom . Indeed, if the lesion and its borders can be seen, and that lesion explains the patient’s semiology and EEG findings, the case immediately becomes much more straightforward for the neurosurgeon. Common neuronavigation planning software is used and the lesion is found and resected completely in most cases, notably if it does not overlap eloquent cortex. Some neurosurgery teams also use ECoG for epilepsy surgery even when there is a visible lesion, as some tumors, like gangliogliomas, can blend subtly into what appears to be a normal brain . In addition, benign brain tumors, cavernous malformations, and some cases of MTS can be associated with FCD and more extensive neocortical involvement . However, the use of ECoG for tumor resection remains somewhat controversial or at least not widely utilized, and all the limitations of ECoG listed above also apply here .
Pathologies That are Only Partly Seen on Magnetic Resonance Imaging
For the most part, if a lesion can be seen on MRI, there is a very good chance that the neurosurgeon will be able to remove it completely, making a patient seizure-free. The caveat to this is the fact that some lesions can usually be seen to some degree, but their borders can extend well beyond what is resolved by even 3 T MRI . These include, in particular, MCD, such as FCD Type IIb, as well as tubers of tuberous sclerosis . These cases are obviously more challenging because the surgeon cannot rely on routine imaging and neuronavigation to decide where to stop the resection. Some data suggests that PET can help in this regard as the so-called functional deficit zone delineated by PET can extend beyond the visible MRI lesion . Additionally, resection of the lesion based on PET-delineated borders improves seizure freedom. In fact, one of the groups who are proponents of this approach has stated that this has replaced the need for SEEG in many of their cases . These authors reported that postsurgical seizure freedom achieved, using intraoperative neuronavigation to resect lesions delineated by a combination of MRI and PET, reaches greater than 90% with a mean follow-up of over 5 years; we have seen similar results in recent years .
Pathologies That Cannot be Seen on Magnetic Resonance Imaging
The real challenge remains for epileptogenic pathologies which cannot be seen at all on MRI, but for which eventually some of the other presurgical evaluation modalities (PET, SPECT, MEG, EEG-fMRI, and intracranial recording) are concordant enough to delineate a hypothesized lesion with a possible epicenter and potential borders . These cases usually require intracranial recording to determine the SOZ . In our case, this is almost exclusively done using SEEG these days. The potential borders can come from a retrospective examination of the patient’s PET scan, and/or combinations of HFOs (fast ripples in particular), and/or areas of early spread (see above) at the end of the presurgical evaluation . Then, this hypothesized multimodal construct must be conceptually resolved and formulated into a virtual 3D object for resection. A 3D model of the patient’s head and the virtual EZ object is generated using the neuronavigation software ( Fig. 5.2 ), and after registering the patient’s head with the same software and checking its accuracy, a hand-held probe can track the object’s position and surrounding anatomy in real-time . Then, even though the lesion cannot be seen per se on the MRI, the surgeon commits to finding and following this 3D virtual object in real-time during the surgery, by continually monitoring the current working location and borders with a neuronavigation probe linked to the corresponding software.

Of course, the limitation of this simple neuronavigation approach is that there can be some shift of the brain parenchyma of interest at several steps during the procedure . This can occur to some degree just from positioning the head in a slightly different position than during preoperative MRI . This can also occur if mannitol is given during the craniotomy, upon opening the dura, upon egress of CSF, but most of all, and most importantly, when part of the resection has been completed which also depends on the patient’s head positioning and gravity. For example, if a patient is positioned directly supine, with the head straight up and down, with the eyes facing the ceiling, and a part of the posterior frontal lobe is removed, the anterior frontal pole will undoubtedly fall backward and lateral to some degree. This can be a problem if resection is still required anteriorly, because the true anatomic anterior border has now been displaced posteriorly, but remains anterior on the virtual object on the neuronavigation monitors. Understanding the anatomy of the planned resection area along with careful step-by-step planning can help to avoid these problems to some degree. For example, if the plan was to take a resection to the lateral pia of the superior frontal gyrus, and this gyrus was marked at the surface before the resection was started, then the surgeon would still know where the superior frontal gyrus was after the brain had shifted. However, removing a part of the superior frontal gyrus itself can be trickier because no true anatomical border (no sulcus) will be there to double-check the location. It can be helpful to reorient oneself in such a situation; hence one reason for the development of intraoperative MRI (iMRI).
Intraoperative Magnetic Resonance Imaging
iMRI was first used and popularized to optimize the extent of resection for brain tumors . It made perfect sense to use this visual tool to assist in the resection of lesions well seen on MRI. Quickly, this technology was adopted for epilepsy surgery, but as stated above the utility of iMRI for epileptogenic pathologies is highly dependent on whether the pathology at hand can be seen or not seen on MRI . We previously tested the ability of 3 T iMRI-based neuronavigation to improve postsurgical seizure freedom versus the performance of epilepsy surgery with neuronavigation but without the use of iMRI . We stratified the cases in terms of whether the EZ was well-defined (tumors and cavernous malformations) or poorly defined (MCD ). The use of iMRI improved the 2-year seizure freedom from 83% to 100% for well-defined cases. However, for poorly defined cases, 2-year seizure freedom was 50% when iMRI was not used, 33% when a postdissection iMRI was performed (i.e., at the end of the case, when the bone and skull were closed completely, but the surgeon could reopen if felt to be necessary), and 57% when an intradissection iMRI was performed (bone flap not replaced and skin only temporarily closed, which could be rapidly reopened); these outcomes were not statistically significantly different from one another .
Of course, it makes sense that a visual tool like iMRI would not improve the resection of pathologies that cannot be seen with this imaging modality, at least in the way it was used in our study described above, without a consistent strategy to verify that the entire multimodal virtual object had been resected. Presently, we always use iMRI for the surgery of poorly defined focal epilepsy cases. This is based on the approach that a virtual 3D construct is designed based on the most relevant presurgical evaluation for the particular patient at hand. Procedural planning formally relies on this construct, which is formally followed during the surgery in an attempt to resect the EZ completely within reason (i.e., without encroaching into the eloquent cortex). When we believe it is resected completely, we perform an intradissection iMRI, and based on the intradissection iMRI, we decide whether the EZ and its borders have been fully resected. If not, the patients come out of the iMRI suite and the temporary closure is reopened to continue the surgery using the new iMRI information/location of the residual EZ and borders. When the residual has been removed, another iMRI is performed to again check if the resection is complete before closing permanently. We feel this has been very helpful, but the most important aspect of this is not the iMRI nor the neuronavigation or other components of the intraoperative workflow, but the multimodal presurgical evaluation that allows us to design the “best hypothesis” to the best of our current abilities .
We recently published our new 3-tier presurgical evaluation protocol specifically for cases of poorly defined focal epilepsy in children . This strategy includes 3T-MRI, PET, SPECT, and MEG in tier-1, then EEG-fMRI and voxel-based postprocessing of MRI in tier-2 if needed, and finally intracranial recording in tier-3 if the first two tiers did not produce a strong hypothesis as per team consensus at our multidisciplinary comprehensive epilepsy conference. Depending on the case, various components of this extensive presurgical evaluation protocol go into conceptualizing, formulating, and designing the 3D virtual EZ object in the surgical planning software, which is then strictly followed during the surgery and intradissection iMRI is used to determine if the virtual EZ object had been removed completely. The challenge to this is that unless the neurosurgeon gives this plan to the neuroradiologist beforehand and the neuroradiologist has a good understanding of the 3D nature of the plan and how it corresponds to the patient’s structural anatomy, the neurosurgeon is usually left to his/her own devices to interpret whether the virtual EZ has been completely removed or not. Strong teamwork and communication with our neuroradiology colleagues at our center have allowed this system to work quite well, however, the interpretation is more subjective than what would occur if an avidly enhancing brain tumor was the target of the resection, and it can be difficult to incorporate such intraoperative results into long-term research studies. However, we showed that upon using this approach, on average 11% greater volume of the suspected EZ is removed using intradissection iMRI to tell us that further resection is needed .
With our current approach -which includes the use of iMRI guidance to follow the multimodal virtual surgical plan along with our 3-tier presurgical evaluation strategy- we have improved our 2-year seizure freedom rate from 27% for poorly defined cases to 85% in recent years, but we have not used “gross total resection” or “complete resection” as variables in retrospective research studies of these cases . This is because, by definition, we cannot say if poorly visualized or invisible EZs have been removed completely and this would be nearly impossible to discern from a retrospective chart review. We can only speculate that complete removal is achieved if a patient becomes seizure-free for many years. The greatest limitation of this approach is that no matter how precisely and completely the virtual hypothesized EZ is removed, the postsurgical outcome for the patient will only be as good as the hypothesis itself. In other words, even a surgical plan based on our best hypothesis might leave epileptogenic histopathology at the borders of the resection. What the neurosurgeon really needs is an intraoperative, real-time method of assessing the pathology at the tips of his/her surgical instruments and checking whether the borders of the resection are clean of this histopathology at the end of the case.
The Future: Intraoperative Dedicated Epileptogenic Zone Border Sampling and Indirect Histopathology Assessment
For the poorly defined cases of focal epilepsy, it would be ideal if the neurosurgeon could quickly and easily send multiple biopsies from the epicenter of the hypothesized EZ and from the potential borders and get immediate answers that could guide the surgery. Of course, this is not possible for many reasons. First, the pathology in question in these cases is usually FCD, where a diagnosis cannot be made on smear or touch prep or even on a frozen section of poor architectural orientation. Even a well-preserved and well-oriented specimen of intact pia, full cortex, and underlying white matter for hematoxylin and eosin staining of a perfectly cut frozen section would take an extremely time-consuming detailed exploration by the most experienced pathologist, and this would be for only one small sample ; the same would need to be done at countless borders and at multiple depths. This of course is not at all feasible during the time constraints of open surgery. In fact, even if a small number of specimens were taken from various borders, it would take days to get final results for all of these samples.
While not useful to guide the surgery at hand, this different type of “intraoperative adjunct” involving taking multiple dedicated specimens from different borders and depths can be useful if the patient were to have a recurrence or persistence of seizures following surgery, which we know is unfortunately very common. With such an approach we could target the side (or sides) of the resection cavity that appeared to show the greatest concentration of abnormal cells. We have been doing this for almost 10 years at our center. In addition to sampling the hypothesized epicenter, we routinely take multiple dedicated, well-labeled specimens (ranging from 6–18 specimens in our experience thus far) from the various hypothesized borders, within reason. These specimens often include several anterior, superior, posterior, inferior, and superficial samples as well as samples taken halfway to the bottom of the sulci and at the depth of the sulci. The specimen results invariably show a gradient of histopathology usually with full-blown or “frank FCD” at the epicenter, and borders which are either normal or show only “rare dysmorphic neurons.” We like to see a result of rare dysmorphic neurons or normal tissue (of course without causing the patient neurological problems) at these borders, which we believe signifies that we have gotten to the end of the epileptogenic pathology at least in that direction, but in some cases, frank FCD is diagnosed in these border specimens, and we must hope that this is below some critical threshold to disallow the production of seizures in the future. However, if the seizures persist or recur, we will already have at least one refined hypothesis for the location of the EZ, that could be either addressed with another resection directly or provide another opportunity to implant SEEG electrodes to better define the extent of the residual histopathology. See the illustrative case, Fig. 5.3 . Of course, if this abnormal tissue resides adjacent to the eloquent cortex, another palliative approach such as callosotomy, vagal nerve stimulator (VNS), deep brain stimulation, or responsive neurostimulation could be entertained , unless the child is very young and could overcome a motor or language deficit reasonably well or completely with rehabilitation .
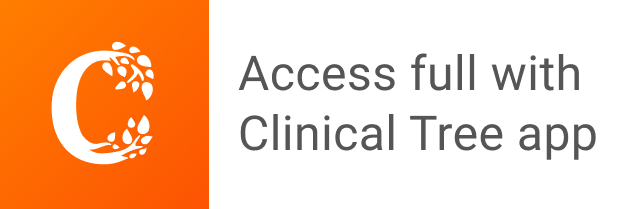