The challenge for treating complex lesions with the aim of minimal surgery-related morbidity has generated an increasing demand for sophisticated intraoperative imaging modalities. Newest generations of intraoperative computed tomography (CT) scanners offer a multitude of hardware and software improvements resulting in higher image quality that further propagates its everyday usage in the interdisciplinary operative setting. This article describes workflow and applicability of intraoperative CT scanning in cranial neurosurgery, in subspecialties like skull base or vascular neurosurgery, and its advantages and limitations.
Key points
- •
Modern intraoperative computed tomography (CT) systems provide similar image resolution as firmly installed CT scanners of radiology departments, including the possibility of performing CT angiography, CT perfusion imaging, and software for artifact suppression.
- •
In skull base surgery, where anatomy is often distorted, the fusion of preoperative MRI and positron emission tomography (PET) data with the intraoperative CT data might provide valuable information for anatomic landmarks and extent of tumor resection.
- •
In vascular surgery, intraoperative CT angiography allows one to define the parent vessel anatomy after clipping, whereas intraoperative CT perfusion enables one to show distant critical perfusion impairment in case of a clip stenosis.
- •
Given its cost-effectiveness, interdisciplinary versatility, the simplicity and velocity of the intraoperative workflow, modern intraoperative CT offers an affordable and reasonable technology, which can be easily installed and implemented.
Introduction
Modern neurosurgical treatment strategies include modalities for intraoperative imaging. Aside from intraoperative ultrasound (iUS), fluorescence angiography (FA), and digital subtraction angiography (DSA), fluorescence-guided resection using 5-ALA (FGR) and intraoperative MRI (iMRI), new technical advances in hardware and refined software solutions have brought intraoperative computed tomography (iCT) back into the field.
iCT was introduced into modern neurosurgery in the early 1980s and has gained attraction since then. First reports showed a general feasibility of this technique, but greater acceptance was not achieved because of initially insufficient image quality, reduced soft-tissue contrast, and image distorting artifacts attributable to hardware components of patient positioning devices. In the mid-1990s, intraoperative MRI (iMRI) was claimed to be the imaging method of choice for intra-axial lesions of the central nervous system. Although limited to certain indications, widespread iMRI usage is nowadays also hampered by the need for magnetic shielding of the room requiring major construction, the need for dedicated MR-compatible operating room equipment, and the high costs of the installation and maintenance without clear proof of cost-beneficial results. Newest generations of iCT scanners offer a multitude of hardware and software development, with largely improved image quality facilitating routine application in an interdisciplinary setting. This article describes the workflow and applicability of intraoperative CT scanning in cranial neurosurgery with focus on the authors’ experience in skullbase and vascular neurosurgery including advantages and limitations.
Introduction
Modern neurosurgical treatment strategies include modalities for intraoperative imaging. Aside from intraoperative ultrasound (iUS), fluorescence angiography (FA), and digital subtraction angiography (DSA), fluorescence-guided resection using 5-ALA (FGR) and intraoperative MRI (iMRI), new technical advances in hardware and refined software solutions have brought intraoperative computed tomography (iCT) back into the field.
iCT was introduced into modern neurosurgery in the early 1980s and has gained attraction since then. First reports showed a general feasibility of this technique, but greater acceptance was not achieved because of initially insufficient image quality, reduced soft-tissue contrast, and image distorting artifacts attributable to hardware components of patient positioning devices. In the mid-1990s, intraoperative MRI (iMRI) was claimed to be the imaging method of choice for intra-axial lesions of the central nervous system. Although limited to certain indications, widespread iMRI usage is nowadays also hampered by the need for magnetic shielding of the room requiring major construction, the need for dedicated MR-compatible operating room equipment, and the high costs of the installation and maintenance without clear proof of cost-beneficial results. Newest generations of iCT scanners offer a multitude of hardware and software development, with largely improved image quality facilitating routine application in an interdisciplinary setting. This article describes the workflow and applicability of intraoperative CT scanning in cranial neurosurgery with focus on the authors’ experience in skullbase and vascular neurosurgery including advantages and limitations.
Methods and technology
A single-room iCT scanner (Siemens SOMATOM Sensation 40 Open with a 40-slice sliding-gantry, Siemens Healthcare, Forchheim, Germany) with a 40-slice sliding gantry was available for neurosurgical procedures and trauma surgery at the authors’ department from January 1, 2006, to August 31, 2014. Since September 1, 2014, a SIEMENS HEALTHCARE SOMATOM with a sliding gantry is installed in between 2 neighboring operating rooms and can be used in simultaneous neurosurgical procedures (double room CT scanner). The sliding gantry can be moved into both operating rooms on rails, and the maximum travel is 600 cm. The patients are positioned on carbon-made radiolucent surgical tables. The gantry is 197.9 cm high and 238.0 cm wide; the diameter of the gantry aperture is 78 cm, and the weight of the scanner is 2850 kg. The needed power including the water cooling system is 100 kW, and the heat dissipation is 1300 W. The iCT scanner is scalable from 20, 40, 64, up to 128 slices depending on the application.
Pre- and intraoperative imaging in cranial surgery was performed using a collimation of 128* 0.6 mm at 120 kV, 410 mAs, a pitch of 0.55, and a rotation time of 1.0 seconds. Reconstructions were done with slice thicknesses of 3.0 mm. If necessary, contrast medium was administered via an automated infusion pump using a weight-adapted contrast agent injection protocol followed by a saline chaser (1.4 mL of contrast agent per 1 kg of body weight [Imeron 300; Bracco- Altana- Pharma, Konstanz, Germany], respectively, 0.42 g of iodine per 1 kg of body weight at an injection rate of 0.035 body weight mL/s followed by 100 mL of saline at 0.035 bodyweight mL/s). For the application of contrast agent, the injector is connected to a central line or at least 10-gauge lumen peripheral venous catheter. Monitoring of the whole procedure is secured by direct visual contact through a lead glass window from a directly neighboring room to get a visual control of all parts of the operating room.
For CT angiography (iCTA), a collimation of 40 0.6 mm was used at 120 kV, 300 mA-s, a pitch of 0.55, and a rotation time of 1 second. Contrast agent was injected with an automated infusion pump using a dedicated protocol (1.35 mL of contrast agent per 1 kg of body weight [Imeron 300], respectively, 0.4 g of iodine per 1 kg of body weight at an injection rate of 4.0 mL/s followed by 100 mL of saline at 6.0 mL/s). For image reconstructions, a 1.0 mm slice thickness and 0.75 mm increment were used. Multiplanar reconstructions, maximum intensity projections in axial, coronal, and sagittal orientations, and reconstructions using a volume rendering technique were used.
For cerebral perfusion measurements (iCT-perfusion), a standard contrast agent injection protocol was used and 1 slice scan was performed every second (7 in total) over a period of 40 seconds using 80 kV and 180 mA-s with a collimation of 32 * 1.2 mm. Perfusion scanning started 5 seconds after injection of 50 mL of contrast agent (Imeron 300) at 7 mL/s and a saline flush of 50 mL at 7 mL/s. After acquisition, standard perfusion analysis was performed using a standard workstation (Syngo MMWP; Siemens Medical Solutions, Erlangen, Germany), and color-coded parameter maps of cerebral blood flow (CBF), cerebral blood volume, and time to peak were created. Scans were performed using a collimation of 24 1.2 mm at 120 kV, 240 mA-s, a pitch of 0.9, and a rotation time of 1 second, using automated dose-modulation software. Multiplanar reconstructions with a 3-mm slice thickness were calculated in axial and sagittal orientations.
Results
Work Flow
After final positioning of the patient in the operating room, a ‘safety check’ is always performed at the beginning of the procedure. Hereby the gantry is moved over the patient in order to avoid any possible collision during the scanning procedure. A special anticollision system prevents any conflict in case the movement of the gantry is obstructed. As the gantry moves over the operating room table, no transfer of the patient to another couch is necessary. The position of the table for the safety check is stored within the operating room table system so that later on, for intraoperative scanning, the table’s position can be reset from any tilting that may have been done in between. All possible positionings including complex ones like park bench were feasible; the only exception for intraoperative scanning is the semi-sitting position. By far most frequently, supine position was used in cranial procedures with iCT, iCTA or iCT-perfusion.
The mean intraoperative examination time for vascular lesions including 3-D CT-angio (iCTA) and a iCT-perfusion was 12 min including 3 min for additional draping/undraping of the patient for the scanning procedure, 1.5 min for image acquisition and 3 min for reconstruction of the data set. A specialized x-ray technician is responsible for contrast agent injection and acquisition of imaging data as well as an attending neuroradiologist for image calculation and interpretation. Simultaneously, the neurosurgical team is taking care of the draping/undraping of the patient as well as any re-positioning of the table position.
Radiation Exposure
The highest radiation exposure for intraoperative CT-scanning was obtained in CT angiography (including CT perfusion studies). Here, the mean effective dose of CTA and CT perfusion together was 3.69 mSv. This is comparable to 3.6 mSv, which is the value typically required for a 4-vessel catheter angiogram but does not include a perfusion study.
Skullbase Neurosurgery
Despite its general applicability in neurosurgery, in our hands for cranial procedures the main use of intraoperative CT scanning is in skull base and neurovascular surgery. In skullbase lesions involving orbit, paraorbital structures and the optic nerve, resection is the most promising and most effective therapeutic approach with regard to preservation or restoration of visual function in most cases. However, maximum radical operative approaches increase the risk of postoperative functional deficits due to the involved neural structures in a tumor-distorted anatomy. Therefore, targeted operative (complete or partial) tumor removal is an important part of modern interdisciplinary treatment concepts that involve operative as well as radiotherapeutic or medical treatment concepts, thereby optimizing both functional results and tumor control. The iCT can be used in these cases for both iCT-guided neuronavigation as well as intraoperative assessment of extent of resection ( Fig. 1 ).
Neuronavigation
Preoperative cranial MRI was used as principal imaging modality in all cases for image guided surgery. High-resolution T1-and T2-weighted imaging in 3 planes was performed on the day before surgery. A 68 Ga-DOTATOC-PET scan, obtained in cases of extensive and/or recurrent meningioma was integrated in order to evaluate the extent to which osseous structures were affected by the tumor. The preoperatively obtained imaging data was fused using the navigation software ( Fig. 2 ). A ceiling-mounted frame-less infrared-based NNS (Vector Vision, BrainLab) connected to the iCT scanner was used during all operations, allowing direct transfer of the data for automated registration. The registration accuracy was then ascertained by touching 6 superficial anatomic landmarks with a non-sterile indicator tool. If the NNS registration accuracy deviated more than 1.5 mm (as calculated), the co-registration process was repeated. In our study, we found the neuronavigation successfully used in all cases. Data were fused with preoperative obtained MR-images or 68 Ga-DOTATOC-PET-CTs. Baseline scanning and NNS referencing was performed within 15 minutes in all cases, radiation exposure was 62.7 ± 3.2 mGy (mean ± SD).
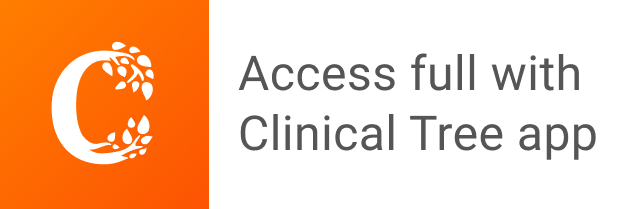