Introduction
Intraoperative imaging has played a substantial role in the history of transsphenoidal surgery. Cushing’s discontinued his use of transnasal pituitary surgery mainly because of limited visibility during the operation. Besides the microscope, several imaging methods contributed to the high standards of transsphenoidal surgery of today. X-ray fluoroscopy was the first intraoperative imaging modality introduced in 1965 by Hardy; this technique allowed tracking of surgical instruments on the bony structures of the cranial base. In 1984 gas cisternography was described as a method to estimate tumor resection. In 1993 the first two series of intraoperative CT-controlled transsphenoidal surgery were published. Although this imaging method allows acceptable resection control, it does not seem to be widely accepted, probably because of limited image quality on coronal sections and poor delineation of adjacent structures, long scanning times, ionizing radiation, patient transfer and positioning problems, and beam hardening artifacts. Intraoperative ultrasound used during transsphenoidal procedures was described first in 1994 as a valuable tool detecting and localizing microadenomas. Intraoperative transsellar color-Doppler ultrasonography was found to be useful for resection control, visualization of cavernous sinus invasion, and concomitant aneurysms in a series of 23 patients in 1998. In a few cases transcranial ultrasonography was applied through a frontal burr hole. The interpretation of intraoperative sonograms has a significant learning curve; ultrasonography in the skull base region is limited by artifacts created by the osseous structures around the pituitary, and resection control may be difficult to estimate due to artifacts created by blood and air bubbles in the resection cavity. The field of view of intraoperative ultrasound is sectorlike restricted, although recent developments, such as three-dimensional ultrasound, can possibly overcome this problem. Some disadvantages also result from the fact that one can only see where one points the ultrasound probe. In 1999 came publication of the first application of intraoperative MRI (iMRI) for transsphenoidal adenoma resections. The system pioneering iMRI, with a double doughnut design, precludes patient positioning necessary for transsphenoidal operations; this may explain to some extent why transsphenoidal surgery was not more frequently performed in this system. Other iMRI configurations were developed and applied more frequently for pituitary surgery.
Imaging Modalities Applied to Transsphenoidal Surgery: |
---|
1965: X-ray fluoroscopy |
1984: Cisternography |
1993: Intraoperative CT |
1994: Intraoperative ultrasound |
1999: Intraoperative MRI |
Current Trends and Technology
Among the different imaging modalities mentioned previously, iMRI, although in its infancy, seems to be the most promising, especially in transsphenoidal surgery, because of its superior image quality with high soft tissue contrast and image acquisition in 3D planes.
Magnetic resonance imaging technology uses the principle of nuclear magnetic resonance to visualize the human body. A proton within the nucleus of an atom possesses charge, mass, and spin angular momentum. The rotation or spinning of a proton around its axis generates a magnetic field that makes the proton behave like a small bar magnet with a north and south pole oriented along the spin axis. When protons are placed in a much stronger magnetic field, their spin axes are aligned parallel to the direction of the external magnetic field. In this environment, the application of a radiowave pulse causes some of the protons to absorb energy and momentarily shift out of alignment with the magnetic field or resonate. When the radiofrequency pulsewave is turned off, the protons return to their original alignment parallel to the external magnetic field and release the stored energy as a weak radio frequency signal. Useful MR images of the human body can only be obtained if the nuclei targeted are ubiquitous in biological tissues. Consequently, MR imaging is usually directed at hydrogen nuclei. The resultant images are based on the distribution of water and lipids within the tissues. This technology was applied for the first time in 1973; in 1977 the first MR scanner became available.
Types of Magnets
Three types of magnets are currently used in MR imaging: permanent, resistive, and superconducting. Permanent magnets are constructed from permanently magnetized material and are relatively inexpensive to install, operate, and maintain. However, these magnets are sensitive to changes in temperature. Resistive magnets consist of many coils of wire wrapped around a bore. An electric current is passed through the wire to generate a magnetic field. These magnets are less costly to produce than superconducting magnets and, unlike permanent magnets, the magnetic field can be extinguished by disconnecting the electricity supply. Very large currents (100 to 150 amperes) are required to generate a magnetic field, which makes the cost of powering and cooling magnets with field strengths greater than 0.3 tesla prohibitive. Superconducting magnets are the most commonly used in MR scanners. The design is similar to that of a resistive magnet except that the coiled wire is supercooled by submersion in liquid helium to eliminate electrical resistance and reduce power consumption. Superconducting magnets for human use can generate fields ranging from 0.5 to more than 7 tesla.
iMRI in Neurosurgery
The ideal neurosurgical iMRI system with excellent image quality and good integration into the neurosurgical workflow and environment is not yet developed. Currently we have a choice of high-field and low- or ultra-low-field systems. High field strength goes along with high image quality, high capital investment, and limited integration into neurosurgical workflow and environment. Low field strength implies lower image quality, longer acquisition times, reduced capital investment, and, depending on the design, good integration into the neurosurgical workflow and environment. In choosing the right iMRI system for neurosurgical use, the following questions need to be answered:
- •
Is the increased image quality and faster image acquisition times, especially for T2-weighted sequences, of high-field systems compensating for the high capital investment?
- •
Is the lower image quality, longer acquisition times (T2-weighted sequences) of low-field systems sufficient for neurosurgical guidance and decision making?
Key features related to intraoperative MRI systems to be taken into consideration: |
---|
|
Current iMRI systems |
---|
|
|
|
|
|
|
|
|
Imaging
Image quality is not solely dependent on field strength, but also on strength of the gradients, design of head coils, and MR sequences and acquisition times. A small head coil will generate better resolution in a smaller field of view and vice versa. Future coil designs could include multiple small coils with good image quality also in low-field magnets. Intravenous contrast media also contribute to image quality in contrast-enhancing lesions. In low-field systems, contrast media can be applied as double dose (only in patients with normal kidney function) to further improve image quality. Extravasations of blood during surgery or scanning sometimes creates accumulations of high-signal intensity fluid. The best way to solve this problem is to keep the resection cavity clean by continuous and meticulous hemostasis. This is sometimes difficult, especially in pituitary surgery. Therefore local compression by soft material, for example bone wax in a piece of rubber from a surgical glove, may help. Ferumoxtran, a blood pool contrast agent given 24 hours preoperatively, is currently under evaluation for assessment of brain tumor resections in iMRI. The obvious advantage of this contrast agent is that it is accumulated in neoplastic lesions and does not leak into the resection cavity.
Field of view should be sufficiently large so that not only the lesion itself, but also the surrounding structures are visible. The patient should be easily accommodated inside the magnet, a requirement that was solved in PoleStar N20, but led to difficulties in the previous version N10 for big patients with short necks (in our experience approximately 2% of the patients would not fit into the magnet of the PoleStar N10).
Before an image set can be acquired, the patient or the magnet has to be brought to “ imaging position .” This is an important factor that may contribute considerably to the time needed to acquire an image. There are basically two options: either the patient is transferred to the magnet or the magnet is transferred to the patient for imaging ( Figure 8-1 ).

The dedicated iMRI systems bring the magnet to the patient. The Signa SP iMRI (General Electric) is the only system that does not require (and allow) any changes in position of the patient or magnet for image acquisition. The Neuro II (IMRIS) is moved along a ceiling-mounted crane, obviating the transport of the patient in and out of the magnet. The two Polestar systems, N-10 and N-20, are located below and on the side of a regular OR table and moved up to scanning position within 30 seconds.
The adapted systems are standard diagnostic open MRI systems with specially designed operating tables allowing patient transfer from the surgery position into the magnet. Magnetom Open (Siemens Medical Systems), Gyroscan (Philips Medical Systems), Airis II (Hitachi), and BrainSuite (Siemens Medical Systems and BrainLab) use different ways of transferring the patient into the magnet. The BrainSuite uses a rotating operating table that permits transfer of the patient’s head in the scanner and out of the 5 Gauss safety line for surgery, so standard neurosurgical instruments can be used.
The imaging sequences most often used for pituitary surgery are T1-weighted contrast-enhanced sequences and, to a lower extent, T2-weighted sequences in coronal and sagittal planes. The higher the field strength of a system, the shorter are the image acquisition times ; this applies especially for the T2-weighted sequences. The field of view describes the imaged area of the brain and is limited in the systems with lower field strength. Real-time imaging is provided by specially developed sequences with a high temporal and lower spatial resolution. In transsphenoidal procedures imaging between surgical steps is sufficient. Instruments remaining in the imaging field during scanning, as the nasal speculum, should be MRI compatible. In mid- to high-field systems, either all instruments have to be MRI compatible or the surgical procedure is performed outside the 5 Gauss line of the magnet.
Navigation
All iMRI systems are linked to a neuronavigation system with an accuracy of 1 to 3 mm. Tracking is achieved optically, with passive (light reflecting spheres) or active (LEDs [light emitting diodes]) markers. Some pointing devices are obstructing the view of the operative site and need to be improved in the future. Registration is different in the various systems. In the Signa SP the patient is operated in the imager and therefore no registration of the patient is necessary. In all other systems, the patient or the magnet needs to change position to some extent during surgery, making registration necessary. In the PoleStar N-20 the magnet is registering itself by LEDs on its surface.
Repeated image acquisitions during surgery provide an updated image map for the integrated frameless navigation systems. If any change of the patient’s head position should occur, a new scan should be produced to have an accurate anatomical map. If there are any doubts concerning navigation accuracy, a small piece of bone wax is brought into the surgical field. Its position is visualized by well-detectable signal void and provides a rough estimate of the positional accuracy of the navigation system.
Integration/Ergonomy
Integration of an iMRI system into a neurosurgical operating room and neurosurgical workflow is crucial and related also to the degree of ergonomics that should allow a surgeon to work in a relaxed and comfortable position to keep his or her movements and manipulations easy and soft. Besides the neurosurgical workflow, the acquisition of a new set of intraoperative images should be as easy as possible; it should not be of concern for the neurosurgeon in any way so he or she can use this technology at any time during a surgical procedure. As mentioned previously, the adapted iMRI systems require transfer of the patient or the magnet for intraoperative image acquisition. Depending on the system, the time needed for this transfer to imaging position may require 1 to 20 minutes. The PoleStar systems offer a so-called starshield, a tent filtering radio-frequency noise during imaging. This foldable Faraday tent makes the installation and related costs of a Faraday Cage in the OR obsolete. Although this may be financially attractive, it adds, every time the surgeon wants to scan, an additional 1 to 2 minutes. Scanning times vary from a couple of seconds to several minutes. In transsphenoidal cases typically approximately 4 minutes scanning time is required for T1 scans with 4 mm slice thickness.
Some systems require a system-specific OR table; others work with most conventional OR tables. For transsphenoidal surgery it is mandatory that an OR table can be tilted to an anti-Trendelenburg position during surgery. Changing position in the Signa SP system is not possible and we found working within the double doughnut to be restrictive and tiring, especially as the nurse works in back of the surgeon, who needs to turn around to get the instruments. The neurosurgical access to the patient, which measures in conventional neurosurgery 270 degrees, is also important, although not necessary for transsphenoidal procedures, and not available in all systems ( Figure 8-2 ).

Higher-field systems usually need to be controlled and operated by neuroradiologists and MR technicians, increasing the amount of personnel and running costs. The availability of an iMRI system for surgical procedures, which under ideal circumstances is available 365 days a year and 24 hours a day, is related to the number of MRI-specific personnel needed to run a system. The PoleStar systems are the only ones that can be run by the neurosurgical team itself.
High-field systems require either MRI-compatible surgical instruments or transfer of the patient beyond the 5 gauss line. Low-field systems such as the PoleStar require only those instruments to be MRI compatible which remain in the surgical field during scanning, such as nasal specula or retractors. The additional costs of MRI-compatible instruments should not be underestimated.
Some of the iMRI systems are multipurpose systems, making the system available also for diagnostic imaging. Although this setting may be economically attractive, there are concerns with regard to sterility and availability of these systems for operative work.
Costs
Currently iMRI systems range in cost from approximately $1 million to more than $6 million. Depending on the iMRI system, further costs for radiofrequency shielding are in a range between $150,000 to $400,000. If an institution is planning to invest in iMRI technology in the future, it may be wise to include a Faraday cage during a scheduled renovation of an OR unit. In addition, the costs of constructing a facility to house and support an iMRI unit range from $700,000 to $1 million. “Downtime” of an existing OR during renovation for installation of an iMRI system is also adding to the overall costs. The servicing contracts are typically in a range of approximately 5% to 10% of the purchase price per year. Depending on the system’s requirements, additional running costs for personnel needed to run a system have to be accounted for. MRI-compatible neurosurgical instruments ($20,000 to $200,000), microscope ($150,000), and anesthesia equipment ($70,000) are required in variable degrees in the different iMRI systems and may add up costs considerably.
With regard to the high costs of investment in this technology, it should be taken into consideration that iMRI technology is still in an early phase and rapidly developing. User friendliness, quality, and prices are expected to improve considerably over the coming 5 to 10 years.
Single Costs | |
---|---|
Purchase price: | $1-$6 million |
Radiofrequency shielding: | $150,000-$400,000 |
Construction: | Up to $1 million |
Downtime OR: | Variable |
MRI-compatible instruments: | $20,000-$200,000 |
MRI-compatible microscope: | $150,000 |
MRI-compatible anesthesiologic equipment: | $70,000 |
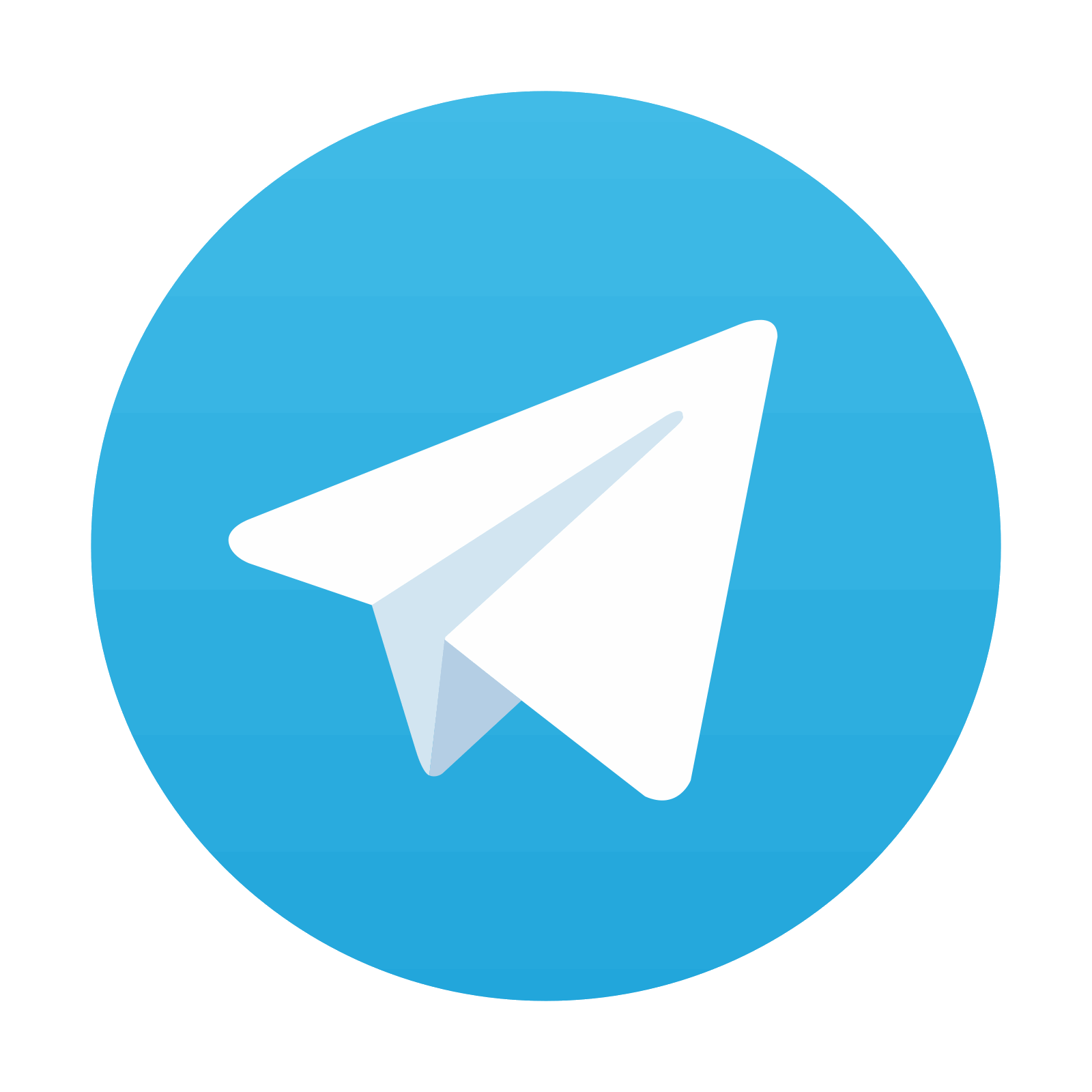
Stay updated, free articles. Join our Telegram channel
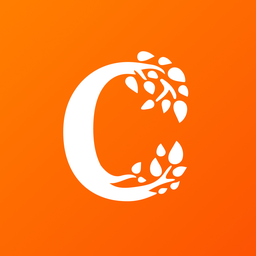
Full access? Get Clinical Tree
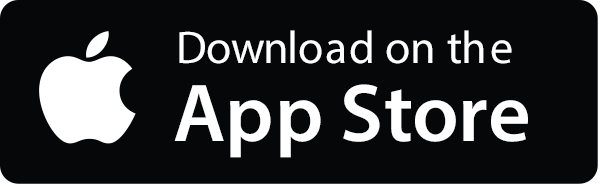
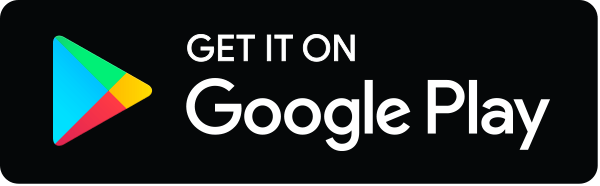
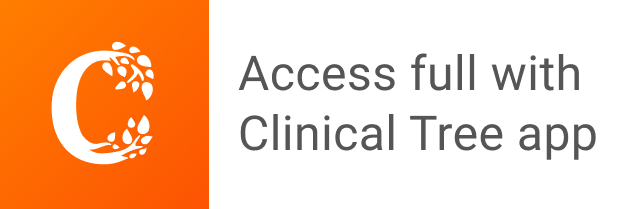