Intraoperative Neurophysiologic Monitoring during Surgery Keywords: somatosensory-evoked potential, motor-evoked potential, visual-evoked potentials, cranial nerve monitoring, intraoperative neurophysiologic monitoring, skull base meningiomas Abstract Intraoperative neurophysiological monitoring (IONM) represents a valuable tool in the management of skull base meningiomas. First, through neurophysiological mapping techniques it is possible to identify, and spare, ambiguous neural structures that could be displaced or encased by the tumor. This is particularly valuable to identify cranial nerves when the anatomy is distorted. Second, the surgical manipulation of cranial nerves, perforating vessels, and brain or brainstem parenchyma may expose patients to substantial risk of neurological injury. In this perspective, IONM provides a continuous functional feedback on the functional integrity of motor, somatosensory, auditory, and visual pathways. Whenever warning criteria for changes in the evoked potentials are reached, the surgeon is informed of an impending injury and can adjust the surgical strategy in order to take corrective measures to reverse or minimize the risk of injury to the nervous system. The combination of both mapping and monitoring techniques provides a multimodality approach, which offers the best chance to avoid neurological deficits. The application of different IONM strategies during skull base surgery must be tailored to the location of the tumor, according to the vascular and neural structures involved. This chapter will critically review the most common IONM techniques and their application during surgery for skull base meningiomas. Surgical management of skull base meningiomas usually carries the risk of postoperative neurologic dysfunctions. The technique used for skull base surgery is based on microsurgical “navigation” inside the basal cisterns through the subarachnoid space in order to avoid or at least to reduce the manipulation of the brain as much as possible, which can cause contusions and/or hemorrhages. Brain arteries and cranial nerves run through the cisternal compartment and can be displaced, encased or even destroyed by skull base lesions. Thus, postoperative neurologic deficits are mainly related to arterial damage and/or injuries to one or more cranial nerves. Different locations and grow patterns of skull base tumors are related to different surgical risks. For example, the removal of an anterior clinoid meningioma that encases the carotid bifurcation and the proximal M1 segment is mainly related with the risk of damage to the perforating branches arising from the carotid bifurcation and/or the proximal part of the M1 segment; the removal of a cerebellopontine angle (CPA) tumor is mainly related with possible injury to the cranial nerves VII and VIII. In the recent past, the standardization of skull base and cranial approaches as well as the wider use of endoscopic surgery has profoundly impacted on skull base surgery. The capability to approach a skull base tumor from its insertion on the dura, where the tumor receives its vascular supply, is a key factor for a successful surgery and, notably, this goal is nowadays reached through minimally invasive approaches. However, despite new technology and keyhole surgery, skull base tumors are still related with significant risk of postoperative morbidity and mortality. Intraoperative neurophysiologic monitoring (IONM) has increasingly gained value as a method to reduce the risk of neurologic injury. IONM evaluates the functional integrity of sensory, motor, auditory, and visual pathways; also, it permits to map ambiguous neural structures in order to define their functional role. Over the last two decades, IONM has become increasingly valuable during supratentorial brain tumor and cerebrovascular surgery. 1, 2, 3, 4 IONM for infratentorial tumors relies on brainstem monitoring and, for skull base tumors, mainly on cranial nerve (CN) monitoring. 5, 6, 7 While monitoring techniques allow to assess the functional integrity of different neural pathways throughout the surgery, neurophysiologic mapping can assist in identifying peripheral cranial nerves that may have been displaced, encircled, or encased by the tumor. The conjunction of both monitoring and mapping IONM techniques provides a valuable feedback to the surgeon during all the steps of the procedure (▶ Fig. 6.1). The aim of this chapter is to provide a description of the IONM techniques that are useful during surgical removal of skull base meningiomas and to give a simple guide for their use in daily clinical practice. Fig. 6.1 Schematic classification of intraoperative neurophysiology techniques in skull base surgery. Left panel: Neurophysiologic mapping allows to identify the functional landmarks in the brainstem as well as peripheral motor nerves. (a) A handheld monopolar probe is used to electrically stimulate the rhomboid fossa or the peripheral nerve. (b) Compound muscle action potentials are recorded from the muscles innervated by motor cranial nerves. Right panel: Neurophysiologic monitoring allows to keep under control the functional integrity of neural pathways (motor, sensory, auditory) throughout the surgery. See the text for further details on each monitoring technique. VII, recording from the orbicularis oris for the facial nerve; IX/X, recording from the posterior wall of the pharynx for the glossopharyngeal/vagus complex; XII, recording from the tongue muscles for the hypoglossal nerve. MEPs, motor-evoked potentials; SSEPs, somatosensory-evoked potentials; BAERs, brainstem auditory-evoked responses. CBT: corticobulbar tract (Modified from: F. Sala, P. Gallo, V. Tramontano. Intraoperative neurophysiological monitoring in posterior fossa surgery. In: M. Ozek, G. Cinalli, W. J. Maixner, C. Sainte-Rose, eds. Posterior Fossa Tumors in Children. Springer; 2015:239–262.) A very useful technique in skull base surgery is the functional identification of oculomotor nerves by direct stimulation. Either a handheld monopolar probe or a bipolar concentric probe, which has the advantage of more focal stimulation, can be used to deliver low-intensity stimuli directly to the nerve. Rectangular pulses of 0.2-ms duration at 1 to 3 Hz and intensity up to 0.5 to 2 mA are generally used. The more focal is the stimulation, the more specific is the response, reducing the risk of activation of nearby fibers due to current spreading. Recordings are obtained by inserting needle electrodes in the muscles innervated by the cranial nerves. For small muscles such as the oculomotor muscles, wire Teflon-coated electrodes may be used as these are less traumatic. Typically, electrodes are inserted in the superior rectus, lateral rectus, and superior oblique muscles to record from the III, VI, and IV oculomotor nerve, respectively. The same technique can be used to localize oculomotor cranial nerve nuclei at the level of the midbrain (▶ Fig. 6.2). Fig. 6.2 Identification of the oculomotor nerve nuclei at the level of the tectal plate. (a) Sagittal (left), coronal (middle), and axial (right) magnetic resonance images of a midbrain cavernoma. (b) One pair of wire electrodes is inserted in the upper and lateral rectus muscles, bilaterally, to record compound action muscle potentials. (c) Initially (time 12.33), direct stimulation of the superior colliculus (left panel) does not elicit any response from the oculomotor muscles innervated by the cranial nerves III and VI. (d) Later on (time 13.41), stimulation from inside the surgical cavity, during removal of the cavernoma, elicits a consistent response (arrow) from the left upper rectus muscles (L III), indicating stimulation of the nearby nuclei. R III, right upper rectus muscle; L III, left upper rectus muscle; R VI, right lateral rectus muscle; L V, left lateral rectus muscle. (Modified from: F. Sala, P. Gallo, V. Tramontano. Intraoperative neurophysiological monitoring in posterior fossa surgery. In: M. Ozek, G. Cinalli, W. J. Maixner, C. Sainte-Rose, eds. Posterior Fossa Tumors in Children. Springer; 2015:239–262.) When ambiguous tissue is encountered during surgery, the tip of the stimulator is placed on the tissue while the oscilloscope displays the recording muscles to determine whether this tissue is functional or, more simply, to confirm the visual identification of the nerve. For the oculomotor nerves one has to consider that compound muscle action potentials (CMAPs) from extraocular muscles are of low amplitude due to the small number of fibers innervated by each axon, when compared to peripheral muscle units. The latency of the response depends on the point of stimulation along the peripheral nerve, being shorter and shorter as the nerve is stimulated more distally, and it ranges between 2 and 5 ms. 8, 9 This technique is often indicated during surgery for meningiomas involving the cisternal, cavernous, or intraorbital segment of the oculomotor nerves. When meningiomas involve the posterior fossa and CPA, mapping of motor cranial nerves V to XII may be valuable. The same stimulation parameters indicated for oculomotor nerve mapping apply. As a general rule, it should be considered that direct stimulation of an intact peripheral cranial motor nerve should elicit a CMAP with as little as 0.1 to 0.3 mA of intensity (▶ Fig. 6.3). Higher intensities may be required either if the nerve is injured or if the nerve fibers are encased in tumoral tissue. For recording, needle or wire electrodes are inserted in the following muscles innervated by motor cranial nerves: the masseter (CN V), orbicularis oculi and oris (CN VII), posterior wall of the pharynx (CN IX), vocal cords (CN X), trapezius (CN XI), and tongue (CN XII). There is some debate whether a threshold difference between proximal (close to the brainstem) and distal stimulation of the nerve retains a prognostic value for postoperative nerve function. For example, during CPA surgery, some authors suggest that a low proximal stimulating threshold is indicative of good prognosis of the facial nerve (FN). 10 However, others 11 have shown that a proximal stimulation threshold less than 0.05 mA is highly specific (90%) but little sensitive (29%), and therefore does not exclude postoperative facial palsy. Fig. 6.3 Identification of cranial motor nerves through direct stimulation of the peripheral nerve. Left panel: Direct stimulation (monopolar handheld probe, intensity 0.2 mA, single stimulus of 0.5-ms duration) of the left hypoglossal nerve (CN XII) at its exit zone from the brainstem (BS). Right panel: Compound muscle action potentials recorded from the left hypoglossal muscles. Somatosensory-evoked potentials (SSEPs) are used to monitor the dorsal column pathway, from the periphery to cerebral cortex. SSEPs monitoring is obtained through the stimulation of the median nerve for the upper extremities and of the posterior tibial nerve for the lower extremities. Because of the different somatotopy at the cord and at the cortex and to the different vascular territories involved in the cortical generators of these potentials, upper and lower SSEPs should be monitored separately. More specifically, the median nerve SSEP would better assess the middle cerebral artery territory and tibial nerve SSEP the anterior cerebral artery territory. The electrical stimulation of the median nerve induces the depolarization that will generate the upper limb SSEPs; the action potential volley travels through the sensory fibers of the dorsal root and reach the fasciculus cuneatus (above T6); beyond the nucleus cuneatus, the action potential decussates (internal arcuate fibers), reaches the contralateral medial lemniscus, and terminates in the ventral posterolateral (VPL) nucleus of the thalamus. Finally, third-order neurons reach the somatosensory cortex and parietal association fields and are processed by cortical scalp leads. The electrical stimulation of the posterior tibial nerve induces the lower extremity SSEPs; the action potential travels through the fasciculus gracilis to the nucleus gracilis; second-order neurons decussate, travel through the contralateral medial lemniscus, and terminate in the VPL nucleus of thalamus. Third-order neurons project to the somatosensory area and parietal association fields and are processed by scalp leads. Scalp leads are placed at CP3 and CP4 with a forehead reference (Fpz or Fz). Parameters monitored are the SSEP amplitude, latency, and central conduction time (CCT), which refers to the transit time from the dorsal column nuclei to the cortex. Baseline values are recorded prior to any significant patient positioning (this will help to detect and correct pressure or traction on the brachial plexus or peripheral nervous system), prior to incision, at the craniotomy and at the beginning of the intracranial manipulation. A standard protocol is then used for decision making in case of SSEP changes; the following warning criteria are usually adopted: decrease of SSEP amplitude greater than 50%, latency delay greater than 10%, and CCT prolongation of greater than 1 ms. Motor-evoked potentials (MEPs) monitoring is used to assess the integrity of the corticospinal tracts (CTs). Two different techniques are available according to the site of the craniotomy: transcranial electrical stimulation (TES), which is used when the motor strip is not exposed and direct cortical stimulation (DCS), which is applied in case of surgical exposure of the motor strip. The former (TES) is by far the most common technique used for skull base surgery, as the motor strip is almost never directly exposed. TES of the motor cortex is usually applied using corkscrew electrodes, which guarantee low impedance. 12 Our routine MEP protocol is based on six electrodes (C1, C2, C3, C4, Cz–1 cm, and Cz+6 cm) according to the 10/20 International Electroencephalography system. Using different montages of stimulating electrodes provides flexibility to optimize elicitation of mMEPs without muscle twitching, which can interfere with surgery. In most cases, C1/C2 is a better electrode montage for eliciting mMEPs in all contralateral limbs. Occasionally, the montage Cz–1 cm versus Cz+6 cm can better elicit mMEPs from lower extremities, offering also the advantage of less intense muscle twitching than other montages. MEP recording is obtained from muscle of the upper and lower extremities using pairs of needle electrodes. TES is considered a safe method, and there are no major contraindications to its use in the clinical setting. 14 However, there is a potential risk of distal activation of the corticospinal tract as far as the brainstem, if too high intensity is used. 15 In such a situation, this may produce a false-negative result (meaning, the patient wakes up hemiparetic in spite of present mMEPs) because the point of CT activation can be distal to the level of a subcortical injury (e.g., due to an ischemic event). For this reason, stimulation intensity immediately above threshold is chosen in order to avoid unnecessary charge load and also to minimize the risk of distal activation of the descending motor pathways. MEP amplitude and latency should be evaluated in comparison with baseline values and through a standard step-by-step protocol in order to exclude anesthesiologic or technical abnormalities as possible causes of changes in the evoked potentials. Although there is still an ongoing debate on what is considered a “significant” MEP change, modifications in amplitude are more relevant than those affecting latency and any amplitude drop, which exceeds 50% of baseline values, should be reported to the surgeon as it may predict an impending injury to motor pathways. 16, 17, 18, 19 In brain surgery, complete loss of MEPs correlate with a permanent paresis and postoperative evidence, at the neuroimaging, of subcortical ischemia. 19 No changes in MEPs usually predict a good motor outcome from early after surgery. However, robust criteria to interpret MEP changes in intracranial surgery are still not well defined and reports referring specifically to skull base surgery are scarce. MEPs are generally more suitable to monitor subcortical areas while SSEPs are more sensitive to ischemic derangements at the cortical level. Therefore, SSEP recording seems inadequate to monitor function in vascular territories supplied by the perforators while it is certainly indicated when cortical ischemia represents the main risk of surgery. From a methodological standpoint, it should be kept in mind that evoked potentials’ recording should be tailored to the location of the tumor and, therefore, to the territory at risk for ischemia. For example, a tumor which encases the anterior communicating artery, anterior cerebral artery, or basilar artery branch would be better monitored using a bilateral tibial nerve SSEPs and bilateral MEP recordings, while for tumors which encase the internal carotid artery bifurcation and M1 segment, contralateral recordings may suffice. Visual-evoked potentials (VEPs) monitoring is used to assess the integrity of the visual pathway in order to prevent postoperative visual deterioration. A light-stimulating device, or goggles, is placed on closed eyelids; flashing light intensity can be adjusted with an electric current ranging from 0 to 20 mA. Each eye is stimulated separately in order to obtain averaged VEP waveforms. Overall, 40 to 100 flashes are recorded to obtain each averaged VEP waveform, with a stimulus average of one flash (40 ms) per second. Recording montage requires five channels, with electrodes placed on the bilateral earlobes and the left occiput, occipital midline, and the right occiput. A small negative potential and a large positive potential at around 100 ms of latency are recorded, and the amplitude of the VEP is defined as the voltage difference between these two potentials. In order to verify the arrival of the light at the retina, needle electrodes are inserted subcutaneously at the lateral canthi for electroretinogram (ERG) recording. It is useful to record at least two consecutive ERG and VEP in order to confirm the reproducibility of the ERG and VEP waveforms after setup and before surgery. 20 Although many attempt have been made in order to improve the reproducibility of VEPs, stable recordings are difficult to obtain and consequently a clear clinical usefulness remains unclear. However, the use of ERG permits the distinction between clinical and technical problems, such as light goggles displacement or inappropriate visual stimulus delivery. Moreover, the improvement in neuroanesthesia, with the widespread use of total intravenous anesthesia (TIVA), has contributed to improve the reproducibility of VEPs. Brainstem auditory-evoked responses (BAERs) monitoring provides data on the auditory pathway. 21 Bilateral ear transducers create alternate compression and rarefaction square wave clicks with duration of 100 to 200 ms and intensity of 70 dB. The stimulation produces a 7-peak wave and each peak is related to a specific sequence of synapsis along the auditory pathway: cochlear nerve (1-degree peak), cochlear nuclei (2-degrees peak), contralateral superior olivary complex (3-degrees peak), lateral lemniscus (4-degrees peak), inferior colliculi (5-degrees peak), medial geniculate body (6-degrees peak), and acoustic radiation (7-degrees peak). BAERs provide information on the general status of the brainstem, especially when manipulation and dissection around it occur during skull base tumor removal. The interpretation of BAERs can be summarized as follows: dysfunction of the eighth nerve proximal to its cochlear end will cause a prolongation of the I to III interpeak interval, attenuation of waves III and V, or both; the latencies of waves III and V increase in parallel, while the III to V interpeak interval remains almost unchanged as long as the auditory pathways within the brainstem are not affected. A disappearance of wave I only may also be indicative of cochlear ischemia secondary to the compromise of the internal auditory artery. Vice versa, if the cochlea is not injured and the damage to the eighth nerve occurs in the CPA, wave I may persist even if the eighth nerve is completely transected. Damage to the lower pons, around the area of the cochlear nucleus or the superior olivary complex, will also affect waves III and V with delay in latency and drop in amplitude. Damage to the brainstem at the level of the midbrain will affect waves IV or V, but not waves I or III. The standard technique for cranial motor nerve monitoring is the evaluation of the spontaneous electromyography (EMG) activity in the muscles innervated by motor cranial nerves. 22 The criteria proposed to identify EMG activity patterns related with nerve injury have been modified through the years. During manipulation of the nerve irritative activity may appear, but it usually stops shortly after the manipulation has stopped. This pattern is generally related with good prognosis for postoperative nerve function. Conversely, neurotonic discharges and especially high-frequency trains usually last longer than surgical manipulation and are related with possible nerve injury. The clinical interpretation of EMG modification has been studied especially for FN during vestibular schwannoma surgery. 23 However, the reliability of free-running EMG for other motor cranial nerves remains controversial; it should be kept in mind that the same electrical silence (no EMG activity) suggesting that no changes are occurring can be observed even after the section of the peripheral nerve; conversely, the irrigation of the surgical field with cold saline can produce some irritative EMG activity. So, although several criteria have been proposed to identify EMG activity patterns indicative of nerve injury, the terminology remains somehow confusing, and convincing data regarding a clinical correlation between EMG activity and clinical outcome are still lacking. 8 This has been particularly disappointing for lower cranial motor nerves in skull base surgery, 24 while the results are more convincing for monitoring the FN in vestibular schwannoma surgery, especially when considering A-train analysis. 25, 26 In recent years, in the attempt to introduce a more reliable technique than free-running EMG for monitoring motor cranial nerves, the principle of MEP monitoring used in monitoring limb muscles has been extended to the muscles innervated by motor cranial nerves. The first reported application for this technique was focused on facial nerve monitoring during vestibular schwannoma surgery. 27 We used a similar technique to monitor CNs VII, IX/X complex, and XII in brainstem surgery. 28 These so-called “corticobulbar” MEPs permit to assess the functional integrity of the entire corticobulbar pathway, from the cortex to the muscle. Corticobulbar MEPs are recorded after TES with a train of four stimuli of 0.5-ms duration at a rate of 1 to 2 Hz and intensity ranging between 60 and 140 mA. The montage used for the electrode is usually C3/Cz for right-side muscles and C4/Cz for left-side muscles (▶ Fig. 6.4). For recording, electrodes are placed in the following muscles bilaterally: orbicularis oculi and oris (CN VII), the posterior wall of the pharynx or vocal cords (CN IX/X complex), trapezius (CN XI), and tongue (CN XII). The major drawbacks reported for this technique are (1) high-intensity TES stimulation with a C3/C4 montage increases the risk of distal activation of the corticobulbar pathways deep in the brain or even at the level of the brainstem or peripheral nerve, therefore, exposing to false-negative results. A single versus train of stimuli protocol can be used to distinguish between centrally conducted and peripherally activated muscle response. 28 (2) The lateral montage (C3/Cz and C4/Cz) can produce muscle twitches in some patients, especially at higher stimulation intensities and these twitches can interfere with surgery thus forcing the surgeon to transiently stop the procedure. By increasing the number of stimuli and reducing stimulation intensity, muscle twitches sometimes can be reduced, while still obtaining a muscle response. (3) Spontaneous EMG activities, which is common during manipulation of cranial nerves, can hinder the recordings of reliable MEPs from the same muscles, therefore compromising the correct interpretation of electrophysiologic signals. If so, to transiently halt surgery may facilitate the disappearance of the firing activity. Fig. 6.4 Continuous monitoring of corticobulbar motor-evoked potentials. (a, left panel) Schematic illustration of corticobulbar motor-evoked potentials elicited after transcranial electrical stimulations at C4/Cz (left-side muscles) and C3/Cz (right-side muscles). Responses are recorded directly from the muscles innervated by motor cranial nerves VII, IX/X, and XII. The entire corticobulbar pathway, from the motor cortex to the muscles, is monitored with this technique (see text for details). (b, right panel) Continuous monitoring of the corticobulbar tracts from the right IX/X and XII cranial nerves following transcranial electrical stimulation delivered at C3(anode)/Cz(cathode) with a train of 4 stimuli, 0.2-ms duration each, at 95 mA. Modified from: F. Sala, G. Squintani, V. Tramontano. Intraoperative neurophysiologic monitoring during brainstem surgery. In: C.M. Loftus, J. Biller, E.M. Baron, eds. Intraoperative Neuromonitoring. McGraw-Hill; 2014:285–297.
6.1 Introduction
6.2 Intraoperative Neurophysiologic Monitoring Techniques
6.2.1 Mapping Techniques to Identify Cranial Motor Nerves
6.2.2 Monitoring Techniques
Somatosensory-Evoked Potentials Monitoring
Motor-Evoked Potentials Monitoring
Visual-Evoked Potentials Monitoring
Brainstem Auditory-Evoked Responses Monitoring
Cranial Nerve Monitoring
Free-Running Electromyography
Monitoring of Corticobulbar MEPs
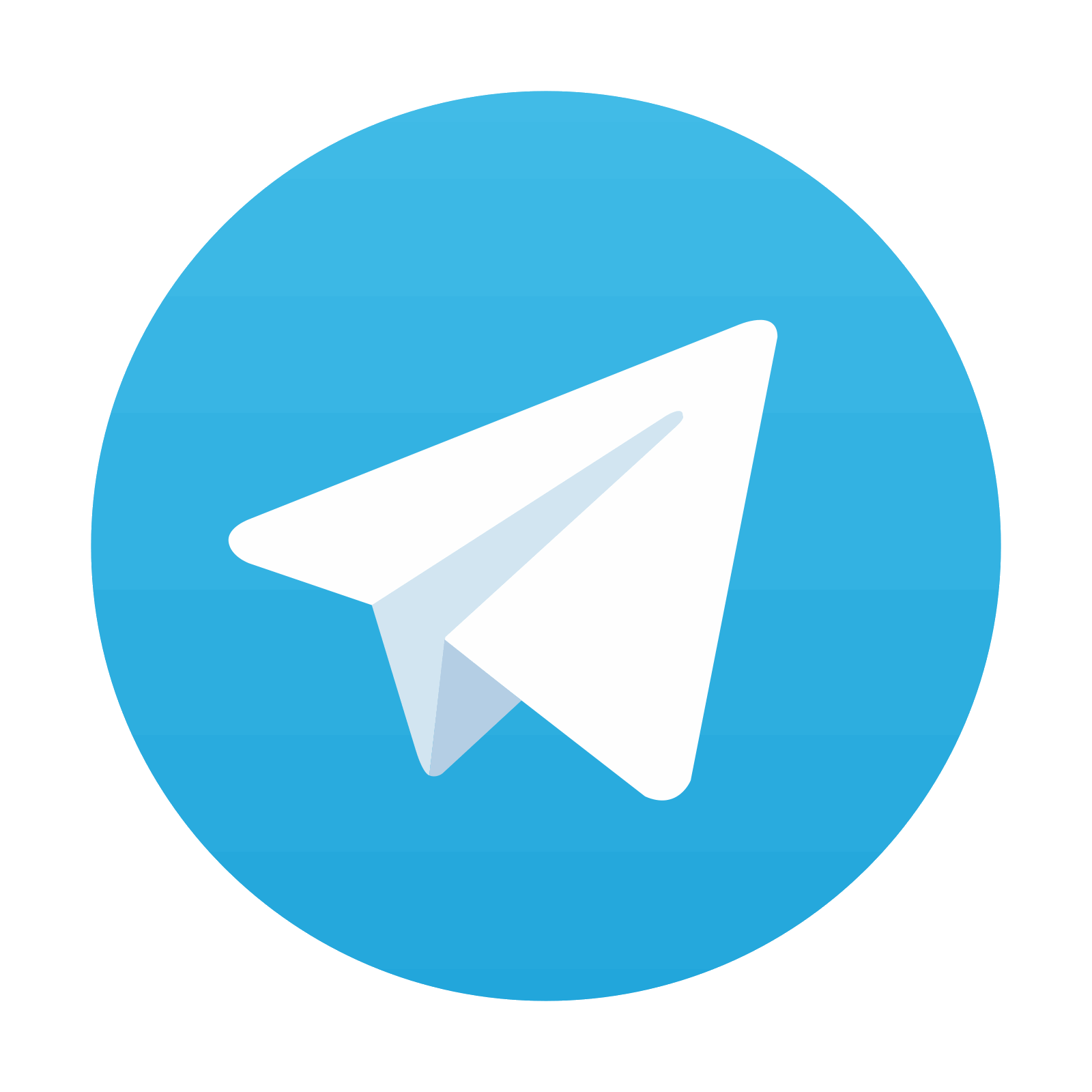
Stay updated, free articles. Join our Telegram channel
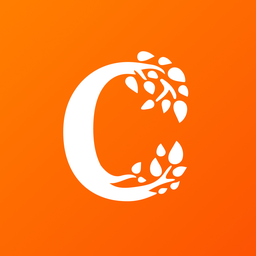
Full access? Get Clinical Tree
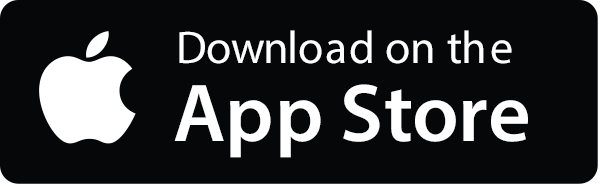
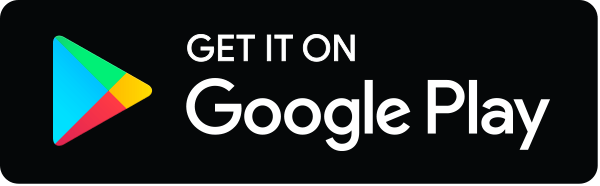