10 Intraoperative Neurophysiological Monitoring during Surgical and Endovascular Treatment of Cerebral Arteriovenous Malformations
Abstract
Intraoperative neurophysiological monitoring (IONM) has emerged over the past two decades as a valuable technique to promptly identify an impending injury to the nervous system, in time for corrective measures to be taken and, therefore, avoid or minimize post-operative neurological deficits. In cerebrovascular surgery, IONM provides a continuous assessment of the functional integrity of various neural pathways, including motor, sensory, auditory and visual, by monitoring evoked potentials.
The treatment of cerebral AVMs can be either surgical or endovascular. Both these treatments expose to the risk of neurological injury and IONM has been increasingly used to prevent, rather than merely predict, brain ischemia during the dissection of the AVM and temporary arterial occlusion. Depending on the location of the AVM and the degree of involvement of cortical and subcortical vascular territories, IONM techniques may include motor evoked potentials, somatosensory evoked potentials, visual evoked potentials, and brainstem auditory evoked potentials. Furthermore, mapping techniques in asleep patients may assist the surgeon to localize the motor cortex and subcortical motor pathways whenever these cannot be identified anatomically, while awake surgery allows to extend neurophysiological mapping to speech and other cognitive areas.
The use of IONM has been applied also during endovascular embolization of AVMs in general anesthesia, when associated with provocative tests. A superselective injection of short-acting barbiturates and/or local anesthetics is done before the embolization of the feeder in order to mimic the effects of the embolization. Based on the results of the provocative test, embolization can either proceed or be abandoned.
This chapter will provide a review of different neurophysiologic monitoring and cortical/subcortical mapping techniques as well as a critical review of the state-of-the art in the use of these techniques in intracranial vascular neurosurgery.
Keywords: cerebral arterio-venous malformations, endovascular embolization, intraoperative neurophysiological monitoring, motor evoked potentials, provocative tests
Key Points
- IONM strategies during the surgical treatment of brain AVMs should be tailored to the location of the AVM and the vascular territories involved. A combination of upper and/or lower extremity MEPs and/or SSEPs, as well as the use of VEPs and BAERs should be considered for each specific case.
- While Penfield’s technique has been the standard method for cortical mapping for several decades, currently the so-called motor evoked potential short-train technique offers several advantages for both cortical and subcortical motor mapping.
- The use of pharmacological provocative tests associated with MEP and SSEP monitoring is a valuable tool for a safe endovascular treatment of brain AVMs in sensorimotor areas under general anesthesia.
- The relationship between the duration of vascular temporary clipping and neurophysiological changes, as well as that between the duration of IONM changes and clinical outcome remains unclear, yet pivotal in understanding how long IONM changes can be tolerated before ischemia progresses to infarction.
10.1 Introduction
Surgical treatment of cerebral arteriovenous malformations (AVMs) is highly demanding, in terms of both operative technique and deep knowledge of anatomy. Furthermore, a high confidence with digital subtraction angiography (DSA) is required. The key point of cerebrovascular surgery, especially in AVM surgery, is to exclude the lesion from the vascular tree and at the same time to preserve the cerebral blood flow (CBF) in the locoregional structures. Arteries feeding the AVM often supply eloquent areas such as the sensory-motor cortex and the corticospinal tracts (CSTs), and a careful evaluation of “en passage” arteries must be performed. Direct visualization of these vessels before and after surgical excision is not enough since hidden perforators can go undetected by surgical inspection alone. In recent years, various techniques have become available to obtain a direct or indirect evaluation of CBF and perfusion. Imaging methods such as intraoperative angiography and, more recently, indocyanine green video angiography provide a realtime evaluation of the vessel patency. Moreover, intraoperative microflow probe1 and Doppler ultrasound provide real-time evaluation of the flow in the insonated vessels. These direct techniques provide qualitative and quantitative assessment of CBF, but they do not provide feedback about the functional consequence of reduced CBF.
Intraoperative neurophysiological monitoring (IONM) allows assessment of the functional integrity of neural pathways during surgery. In cerebrovascular surgery, IONM indirectly provides an assessment of CBF and often allows detection of an impending injury to cortical and subcortical areas. In the recent past, IONM has become a very useful tool in the management of cerebrovascular lesions, especially aneurysms.2,3,4,5,6,7,8,9 Neuro-physiologic monitoring includes various techniques with different characteristics: electroencephalography (EEG) monitoring is useful to detect impending ischemia due to major vessels occlusion and to titrate anesthesia during temporary clipping in aneurysm surgery; somatosensory evoked potentials (SSEPs) provide an evaluation of the dorsal column pathways and their ability to transduce a peripheral stimulus and convey it to the brain; brainstem auditory evoked potentials (BAERs) evaluate the transduction of an auditory stimulus along the auditory pathways (auditory nerve, cochlear nucleus, superior olive, inferior colliculus), from the periphery to the cortex; motor evoked potentials (MEPs) provide an evaluation of the CST and other descending motor pathways, from the cortex to the muscles.
The treatment of cerebral AVMs can be either surgical or endovascular. Both these treatments expose to the risk of neurological injury, and IONM has been increasingly used to prevent, rather than merely predict, brain ischemia.
This chapter will provide a review of different neurophysiologic monitoring (SSEP, BAER, MEP) and cortical/subcortical mapping techniques as well as a critical review of the state of the art in the use of these techniques in intracranial vascular neurosurgery.
10.2 Intraoperative Neurophysiology Techniques
IONM can be essentially divided in two categories: mapping and monitoring techniques. The former provides functional identification of ambiguous neural structures such as the identification of the primary motor area in the case of a deformed functional anatomy due to the presence of a rolandic AVM. However, cortical/subcortical mapping provides only information at a given point in time, but does not allow continuous assessment of the functional integrity of a neural pathway. This latter can be achieved by using monitoring techniques such as SSEP, BAERs, and MEPs.
Unlike tumor surgery, mapping techniques are rarely needed in vascular neurosurgery. On the other hand, monitoring techniques can be used and provide valuable information. Monitoring of SSEPs has been used since the 1980s in aneurysm surgery.2,10,11 Other authors have used a combined SSEP–BAERs protocol,5,12 while MEPs were introduced more recently.6,8,9,13,14
10.2.1 Mapping Techniques Cortical Mapping (► Fig. 10.1)
Fig. 10.1 Schematic illustration of cortical mapping during surgery for brain AVMs. Left-hand panel: direct cortical stimulation (DCS) is performed using a hand-held monopolar probe that delivers a short train of five stimuli (0.5 ms duration each; interstimulus interval, 4 ms; repetition rate, 1 Hz; maximum Intensity, 20 mA). Compound muscle action potentials (CMAPs) are recorded from the contralateral muscles. In this example, CMAPs from the left extensor digitorum (LE) and left abductor pollicis brevis (LA) muscles are recorded following DCS at 12 mA. No response is recorded from the left lower facial (LL) and the left tibialis anterior (LT) muscles.
For cortical and subcortical mapping, the bipolar 60-Hz stimulation technique, which is essentially based on the original Penfield technique, is still widely used in the neurosurgical community.15 A constant-current stimulator generating biphasic square wave pulses with a 60-Hz (50 Hz in Europe) stimulating rate and 1-ms single-phase duration is used. A bipolar electrode with 5-mm spacing between the contact points is used to directly stimulate the cortex for 2 to 3 seconds. Under general anesthesia, starting current intensity is around 4 mA, and is then increased in 2-mA increments up to the point where a motor response is recorded from contralateral muscles. In an awake patient, the threshold is usually lower and movements can be elicited with current as low as 1 to 2 mA.16,17 Visual observation of the muscle activity has been substituted by multichannel electromyography, which appears to be more sensitive.18 In the asleep patient, if at the intensity of 16 to 20 mA no muscle contraction is obtained, the cortical area under investigation is declared not eloquent for motor function, although some patients may have higher thresholds for stimulation. During the application of this technique, it is highly recommended to predispose electrocorticography (ECoG) due to the risk of eliciting clinical or subclinical seizurelike activities (afterdischarge), which may compromise any further neurophysiological assessment. In the case of an intraoperative seizure, cortical irrigation with cold ringer lactate usually stops this activity within 20 to 30 seconds.19
Penfield’s technique, however, has several limitations, which include a higher risk of inducing intraoperative seizures,20 the unfeasibility to perform continuous MEP monitoring due to the prolonged stimulation of the cortex, and a low success rate in children likely due to the immaturity of the motor cortex and pathways.21
With the advent of the MEP monitoring technique developed by Taniguchi et al and Pechstein et al in the mid-1990s, a new strategy became possible to map the motor cortex.22,23 This technique is used to elicit MEPs through transcranial electrical stimulation (TES) but, during cranial surgery where the motor cortex is exposed, it can be used also for performing MEP monitoring directly from a strip electrode on the motor cortex and to perform cortical mapping. The technique accounts for 500-ps square wave impulses in train of five to seven stimuli, a 4-ms interstimulus interval, and constant current intensities up to 200 mA for TES and up to 20 mA for cortical MEP monitoring and motor mapping.
In adults, threshold intensities up to 20 mA are usually considered safe for mapping the motor cortex through monopolar stimulation. Although the risk of inducing intraoperative seizures using the MEP short-train technique is considerably lower than using Penfield’s technique,20 to minimize this risk we still recommend the use of ECoG while setting the intensity for cortical mapping and direct cortical stimulation (DCS) for continuous MEP monitoring. This allows detecting any afterdischarge, which may affect the results of mapping, providing false-positive results. Unfortunately, the threshold for positive cortical mapping is sometimes higher than the threshold for afterdischarges and there is high variability across the cortex within the same subject.24
For a successful cortical/subcortical motor mapping and MEP monitoring, the selection of appropriate muscles to record from is critical. The small hand muscles (e.g., abductor pollicis brevis) as well as the long forearm flexors or extensors have been shown to be good options for upper extremities, due to their richer innervation with CST fibers than more proximal muscles. Similarly, for the lower extremities, the abductor hallucis brevis (AHB) is the optimal muscle because of its dominant CST innervation.25 The tibialis anterior (TA) muscle is an alternative to the AHB. Our standard electrode montage for monitoring mMEPs is the AHB and TA muscle for the lower and the ABP and forearm flexors or extensors for the upper limbs. Finally, it is important to always monitor a muscle that cannot be affected by surgery (typically, in brain surgery, any ipsilateral muscle) as a control modality to exclude changes in evoked potentials not induced by surgery but by anesthesia or technical issues.
During surgery for brain AVMs, the choice of the appropriate muscle recordings is essential, and it should be made based on the functional anatomy of the cortical and subcortical areas related to the AVM nidus and to its angioarchitecture.
Subcortical Mapping
Subcortical mapping provides the identification of the white matter fiber tracts with the aim to preserve them during surgery. Again, either the MEP short-train or Penfield’s technique can be used for subcortical mapping, but some preliminary data26 suggest that the short-train technique delivered through a monopolar probe is the most efficient technique. The CST has received the larger attention in terms of subcortical mapping and correlation with tractography. The lower the intensity of stimulation necessary to localize the CST and elicit a muscle response, the closer the CST. A safety distance between the mapping site and the CST has not been yet unequivocally determined; however, the rule of thumb suggests that each mA of stimulation intensity corresponds to roughly 1 mm of distance from the tracts.27,28 If so, stimulation intensities between 2 and 5 mA correspond to a distance of 2 to 5 mm from the tracts, and are reliable cut-off values for a safe distance from the CST.21,27,29
SSEP Phase Reversal
SSEP phase reversal is a technique that permits to identify the central sulcus and therefore, indirectly, the motor area. A subdural strip electrode is placed over the central region and then SSEPs are elicited through the stimulation of the contralateral median nerve or posterior tibial nerve (up to 40-mA intensity, 0.2-ms duration, 4.3-Hz repetition rate). Recordings are performed from the scalp at Cz-Fz (legs) and C3/C4-Cz (arms). This technique is based on the principle that an SSEP elicited by median nerve stimulation at the wrist can be recorded from the primary sensory cortex and its mirror image waveform can be recorded if the recording electrode is placed on the opposite side of the central sulcus, on the primary motor cortex.30,31 The amplitude of the cortical response recorded at the precentral gyrus is typically positive at around 25 ms (P25), whereas the response visible at the postcentral gyrus is negative at 20 ms (N20).
10.2.2 Monitoring Techniques
MEP Monitoring (► Fig. 10.2)
Fig. 10.2 Schematic illustration of continuous MEP monitoring during surgery for brain AVMs. Left-hand panel: MEP monitoring is performed using an eight-contact strip electrode placed on the primary motor area. The electrode with the lowest threshold to elicit contralateral mMEPs is used for monitoring. Nearby electrodes can be used to obtain mMEPs from other muscles, if needed. The selected electrode (in yellow) delivers short trains of five stimuli (0.5 ms duration each; interstimulus interval, 4 ms; repetition rate, 1 Hz; maximum Intensity, 20 mA), which travel along the corticospinal tract (red arrows) and elicit mMEPs from the contralateral muscles. In this example, a response from the abductor pollicis brevis (APB) muscle is recorded following stimulation from electrode no. 3 at 12 mA. No response is recorded from the tibialis anterior (TA) muscle.
Unlikely from motor cortical and subcortical mapping, continuous MEP monitoring provides an on-line assessment of the functional integrity of descending tracts, rather than their identification at one point in time.
MEP monitoring is used to assess the integrity of the corticospinal pathway. Two different techniques are available: TES and DCS. The former is used in cases in which the motor strip is not exposed with the craniotomy; the latter is preferred in the case of surgical exposure of the motor strip.
TES of the motor cortex is usually applied using corkscrew electrodes, which guarantee low impedance.32 We routinely place six electrodes (C1, C2, C3, C4, Cz-1 cm, and Cz + 6 cm) according to the 10/20 International Electroencephalography system. Using different montages of stimulating electrodes provides flexibility to optimize elicitation of mMEPs without muscle twitching, which can interfere with surgery. In most cases, C1/C2 is a better electrode montage for eliciting mMEPs in all contralateral limbs. Occasionally, the montage Cz-1 cm versus Cz + 6 cm can better elicit mMEPs from lower extremities, offering also the advantage of less intense muscle twitching than other montages. In the case of surgical incision around the central region, leads should be displaced either in front or posterior to the skin flap, but this can require higher current to obtain MEP response with subsequent higher muscle twitches and discomfort for the surgeon.
In the DCS technique, a multicontact strip electrode is placed under the dura over the central gyrus. An electrode at the Fpz serves as cathode. The electrode with the lowest stimulation threshold to elicit a contralateral muscle response is chosen for continuous MEP monitoring. MEP recording is obtained from muscle of the upper and lower extremities using pairs of needle electrodes.33 Muscle twitches following DCS are minimized by the much lower current intensity (up to 20mA) applied to the motor cortex, as compared to transcranial MEPs (up to 200mA).
Stimulation intensity immediately above threshold is chosen for stimulation in order to avoid unnecessary charge load. MEP amplitude and latency should be evaluated in comparison with baseline values and through a standard step-by-step protocol in order to exclude anesthesiological or technical abnormalities as possible causes of changes in the evoked potentials. Although there is still an ongoing debate on what is considered a “significant” MEP change, there is agreement on the fact that changes in amplitude are more relevant than changes in latency and that any amplitude drop, which exceeds 50% of baseline values, should be reported to the surgeon as it may predict an impending injury to motor pathways.6,27,34,35 Transcranial MEPs suffer from intrinsic variability so that threshold criteria per se are of limited value, but—at least in brain tumor surgery—there is evidence that irreversible changes in MEP amplitude correlate with some degree of postoperative motor deficit. Complete loss of MEPs correlate with a permanent paresis and postoperative evidence, at the neuroimaging, of subcortical ischemia.35 No changes in MEPs usually predict a good motor outcome from early after surgery. In between these black and white scenarios, there is a gray zone where robust criteria to interpret MEP changes are still not well defined. This degree of uncertainty is larger in vascular neurosurgery due to the paucity of clinical studies when compared to brain tumor surgery and, in particular for AVMs, there is little amount of data in the literature.
Overall, TES is considered a safe method, and there are no major contraindications to its use in the clinical setting.36 However, if too high intensity is used, there is a potential risk of distal activation of the CST as far as the brainstem.37 In this case, the point of activation may be distal to the level of a subcortical injury (for example, due to an ischemic event) and this may produce a false-negative result (meaning, the patient wakes up hemiparetic in spite of present mMEPs). To avoid this, during intracranial surgery, the use of DCS, whenever feasible, should always be preferred over TES for mMEP monitoring because the intensity required to elicit a response is about 10 times lower (1–20 vs. 30–200 mA), and this minimizes the risk of distal activation of the CST.
MEPs are generally more suitable to monitor subcortical areas, while SSEPs are more sensitive to ischemic derangements at the cortical level. Therefore, SSEP recording seems inadequate to monitor function in vascular territories supplied by perforating arteries, while it is certainly indicated when cortical ischemia represents the main risk of surgery.
From a methodological standpoint, it should be kept in mind that evoked potentials recording should be tailored to the location of the AVM and, therefore, to the territory at risk for ischemia. For example, an AVM fed mainly from an anterior communicating artery, anterior cerebral artery, or basilar artery branch would be better monitored using a bilateral tibial nerve SSEPs and bilateral MEP recordings, while for AVMs fed mainly by middle cerebral artery (MCA) branches contralateral recordings may suffice.
TES MEP monitoring is a valuable method also during the endovascular treatment of cerebral AVMs, as it provides continuous assessment of the functional integrity of motor pathways during the embolization procedure. Provocative tests can be used to mimic the effect of the embolization, as it will be discussed later in this chapter.
SSEP Monitoring
SSEPs are used to monitor the dorsal column pathway, from the periphery to cerebral cortex. The median nerve and the posterior tibial nerve are normally used, respectively, for upper extremities and lower extremities SSEP monitoring. It has to be kept in mind that upper and lower extremity SSEPs should be monitored separately because of the different somatotopy of these tracts and different vascular territories involved in the cortical generators of these potentials. So, median nerve SSEP would better assess the MCA territory and tibial nerve SSEP the anterior cerebral artery territory.
Upper extremities monitoring starts with the depolarization of the median nerve by electrical stimulation in order to produce a synchronous action potential volley through the sensory fibers of the dorsal root that reach the fasciculus cuneatus (above T6). Then the action potential travels through the nucleus cuneatus, decussate (internal arcuate fibers), reach the contralateral medial lemniscus, and terminate in the ventral posterolateral (VPL) nucleus of the thalamus. Lower extremities monitoring starts with the depolarization of the posterior tibial nerve. The action potentials travel through the fasciculus gracilis to the nucleus gracilis; then, second-order neurons decussate, travel through the contralateral medial lemniscus, and terminate in the VPL nucleus of thalamus. Third-order neurons project to the somatosensory area and parietal association fields and are processed by scalp leads. Scalp leads are placed at CP3 and CP4 with a forehead reference (Fpz or Fz). Parameters monitored are the SSEP amplitude, latency, and central conduction time (CCT), which refers to the transit time from the dorsal column nuclei to the cortex.
Baseline values are recorded prior to incision, at the craniotomy and at the beginning of the intracranial manipulation. A standard protocol has then to be used for decision-making in case of decrease of SSEP amplitude >50%, latency delay >10%, and CCT > 1.0ms.
BAER Monitoring
BAER monitoring provides data on the ascending auditory pathway. Bilateral ear transducers create alternate compression and rarefaction square wave clicks with duration of 100 to 200ms and intensity of 70 dB. The stimulation provides a seven-peak wave; peaks are related to the specific sequence of synapses along the auditory pathway: cochlear nerve (I peak), cochlear nuclei (II peak), contralateral superior olivary complex (III peak), lateral lemniscus (IV peak), inferior colliculi (V peak), medial geniculate body (VI peak), and acoustic radiation (VII peak).
Brainstem auditory-evoked potentials can provide useful information on the general well-being of the brainstem, especially during those procedures in which a significant surgical manipulation of the brainstem and/or of the cerebellum is expected. When interpreting brainstem auditory-evoked potential recordings, a thoughtful analysis of the waveform and of their correlation with neural generators provides useful information about the level of the brainstem where EP changes may occur. In summary, dysfunction of the eighth nerve proximal to its cochlear end will cause a prolongation of the I–III interpeak interval, attenuation of waves III and V, or both. The latencies of waves III and V increase in parallel, while the III–V interpeak interval remains almost unchanged as long as the auditory pathways within the brainstem are not affected.
A disappearance of wave I only may also be indicative of cochlear ischemia secondary to the compromise of the internal auditory artery. Vice versa, if the cochlea is not injured and the damage to the eighth nerve occurs in the cerebellopontine angle, wave I may persist even if the eighth nerve is completely transected. Damage to the lower pons, around the area of the cochlear nucleus or the superior olivary complex, will also affect waves III and V with delay in latency and drop in amplitude. Damage to the brainstem at the level of the midbrain will affect waves IV–V, but not waves I or III.
VEP Monitoring
Visual evoked potential (VEP) monitoring is used to assess the function of the visual pathway in order to prevent postoperative visual deterioration. A light-stimulating device is placed on closed eyelids; flashing light intensity can be adjusted with an electric current ranging from 0 to 20 mA by a bath amplifier. Each eye is stimulated separately and averaged VEP waveforms are then obtained. Overall, 40 to 100 flashes are recorded to obtain each averaged VEP waveform, with a stimulus average of one flash (40 ms) per second. Recording montage requires five channels, with electrodes placed on the bilateral earlobes (A +) and the left occiput, occipital midline (Oz), and the right occiput. A small negative potential and a large positive potential around 100 ms are recorded, and the amplitude of the VEP is defined as the voltage difference between these two potentials. In order to verify the arrival of the light at the retina, needle electrodes are inserted subcutaneously at the lateral canthi for electroretinogram (ERG) recording. It is useful to record at least two consecutive ERG and VEP in order to confirm the reproducibility of the ERG and VEP waveforms after setup and before surgery.38 Although many attempts have been made in order to improve the reproducibility of VEPs, stable recordings are difficult to obtain and consequently a clear clinical usefulness remains unclear. However, as previously described, the use of ERG permits the distinction between technical and clinical problems, such as light goggles displacement or preexisting visual dysfunction and VEPs disappearance.
10.2.3 Cognitive Mapping during Awake Surgery
Unlikely from sensorimotor, auditory, and visual function, cognitive functions such as language, memory, and others require the collaboration of the patient and the presence of a neuropsychologist in the operating room. Awake surgery is nowadays very popular and has revolutionized the operative management of brain gliomas, especially low-grade gliomas. As much as the use of awake craniotomy during surgery for brain AVMs is of limited use, still this can be valuable in selected patients.
For cortical and subcortical mapping in awake patients, Penfield’s technique—as described above for motor mapping—is considered the standard of care given that it is considered that a prolonged cortical stimulation is necessary to interfere with cognitive processing.
Berger and Ojemann et al17,39 have developed a classic stimulation paradigm for language mapping. Since the majority of aphasias involve a naming deficit, stimulation evoked anomia (or dysnomia) is considered significant for a language site. In order to confirm localization of Broca’s area, it is then mandatory to check movements of the mouth or pharynx to rule out the possibility of a speech arrest secondary to motor activation of facial, tongue, and laryngeal muscles rather than to specific stimulation of Broca’s site. As a rule, a wide exposure of the cerebral cortex increases the possibility of obtaining positive mapping results and, on average, 20 or more cortical sites need to be mapped to achieve a positive response. Every site is tested three separate times, and two errors out of three are significant for a language site, which should be preserved with a margin of at least 10 mm. Besides language, a number of other cognitive functions can nowadays be tested in the operating room, but this exceeds the goal of this chapter. It should be emphasized, nevertheless, that regardless of the specific functions going to be tested, the use of ECoG is strongly recommended to detect subclinical epileptiform activity, which may be a source of misleading results given that the falsely “positive” cortical mapping is due to the results of afterdischarges rather than the activation of truly eloquent cortex.
10.2.4 Considerations in Pediatric Neurosurgery
Corkscrew electrodes for TES and recordings should be cautiously used in infants with open fontanels and in children with shunt systems to avoid injury due to misplacement of the screw.
In children, higher intensities for either TES or DCS MEP monitoring may be needed, especially in those younger than 5 to 6 years, due to the immaturity of their motor cortex and pathways.21,40 In young children, two opposite factors may affect the threshold to elicit mMEP after TES. The immaturity of the motor cortex and subcortical motor pathways may increase stimulating thresholds. However, this variable is counterbalanced by the thinner thickness of the skull, which should facilitate motor cortex activation at lower intensities because of lower impedance.
With regard to cortical mapping, preliminary data suggest that in children the MEP short-train technique is by far more effective than Penfield’s technique in eliciting a motor response.41
10.2.5 Anesthesia for Neurophysiological Monitoring
The success of IONM is highly related to the anesthesiological management of the procedure. The common inhalational agents such as isoflurane, sevoflurane, and desflurane may affect the reliability of IONM by blocking neural transmission at the synaptic level. Halogenated anesthetics elevate muscle MEP stimulus thresholds and block muscle MEPs in a dose-dependent fashion, and therefore should be avoided.
Total intravenous anesthesia (TIVA) is a preferable choice in the management of anesthesia during IONM. Anesthesia is therefore maintained with a constant infusion of propofol (100–150 pg/kg/min) and fentanyl (usually around 1 pg/kg/h). Nitrous oxide not exceeding 50% can be used. Bolus injections of both intravenous agents should be avoided because this temporarily disrupts SSEPs and MEPs recordings.
Short-acting muscle relaxants are given for intubation but not thereafter, because with full relaxation muscle MEP monitoring and cortical/subcortical mapping are unfeasible. We also try to avoid any partial relaxation because a physiologic variability in the mMEP amplitude across repetitive trials and in the compound muscle action potential response already exists, so that any muscle relaxation would add another variable to the interpretation of motor responses.
10.3 IONM during Surgical Procedures
Surgical resection of brain AVMs is technically challenging. The key point is to cauterize and cut arteries that directly feed the AVM and to spare those that supply the normal brain tissue, especially in eloquent areas. During the surgical procedure, additional radiological details provided by intraoperative angiography may help the surgeon to distinguish between normal brain tissue arteries and pathologic AVM suppliers.42 More recently, indocyanine green videoangiography has been reported as useful tool also in detecting residual nidus during AVM surgery.43 However, intraoperative angiographic studies provide only anatomical data and in some cases it might be still difficult to differentiate between AVM feeders and passing arteries. This is even more crucial when dealing with AVMs in eloquent areas.
The principle of “eloquence” in surgery for brain AVM includes cortical and subcortical areas which can be monitored under general anesthesia and others which require cognitive mapping and, therefore, awake surgery. We will focus on IONM strategies during surgical procedures under general anesthesia as these represent, by far, the most common application of IONM. Some aspects related to IONM in awake patients will be discussed thereafter.
10.3.1 IONM during AVM Surgery under General Anesthesia
IONM in cerebrovascular surgery permits the early recognition of two main problems: impending ischemia due to inadvertent occlusion of vessels or perforators and direct damage to eloquent cortical or subcortical areas. Dissection of cerebral AVMs requires various surgical maneuvers: brain retraction, dissection of brain tissue, temporary artery occlusion. All these steps could possibly induce changes in the evoked potentials. Moreover, inadvertent occlusion ofperforating arteries and vasospasm secondary to manipulation of vessels during subarachnoid dissection are other causes of evoked potential alterations. The role of IONM during the resection of eloquent AVMs therefore becomes substantial. The coupling of temporary clipping of the feeding arteries and the indirect evaluation of the perfusion around the AVM with IONM helps in reducing the risk of cerebral infarction.
Various combinations of the aforementioned IONM techniques have been used to preserve function during surgery for brain AVMs. Chang et al reported the usefulness of SSEP and BAERs in the surgical management of 53 patients (54 procedures) with cerebral AVMs.12 SSEP changes have been reported in five patients (four transient and one permanent): in the four cases with transient SSEP alteration, three patients showed new—but transient—postoperative deficits, and one did not develop any clinical postoperative deficit; the latter patient with permanent SSEP changes developed a prolonged transient hemiparesis lasting 3 weeks. During the surgical procedure, all of the transient SSEP changes were reverted with the removal or adjustment of the temporary clip on feeding arteries or by elevating mean arterial pressure (MAP). One of the 17 patients (6%) monitored with BAERs showed a permanent alteration in BAERs and developed a new permanent neurological deficit after surgery. The authors reported 86% sensitivity for SSEP and BAERs in the prediction of new postoperative transient or permanent deficit, and 98% specificity.
Ichikawa et al have recently described the use of MEP monitoring in AVM surgery in 21 patients.44 In order to address a specific potential risk to motor pathways, the patients were divided in three groups. In the first group, the feeding arteries supplied the CST; the second group included patients at risk of direct injury to the CST due to surgical maneuvers, and the third group had the potential of motor pathways shift due to the AVM location. The surgeon received a warning in case of MEP disappearance or decreasing in amplitude to less than 50% of the baseline level in the course of three or more consecutive recordings. During the surgical procedure, five patients showed MEP changes. Of these, four patients were in group I. In the first patient, MEP disappeared after the occlusion of a draining vein and did not reappear; the patient developed hemiparesis due to a venous infarction of the thalamus and the internal capsule. The other three patients showed transient MEP changes related to temporary clipping in two patients and to bleeding from the nidus in the last one. Only two of the three patients with transient MEP changes developed transient postoperative hemiparesis, which resolved in 2 days; the last patient did not show any postoperative deficits. One patient in group II showed transient MEP decreased amplitude (80%) due to coagulation of fragile vessels around the nidus. In group III, there were no changes during MEP recordings.
Continuous evaluation of the functional activity of the brain in eloquent areas also includes inspection of the visual cortex. Intraoperative monitoring of VEPs may contribute to the preservation of visual function after surgery of the optic pathways (from the optic nerve to the lateral geniculate body). In 1973, Wright et al described the use of VEP monitoring during surgery of intraorbital tumors in order to prevent postoperative visual deficits.45 However, difficulties in obtaining stable VEP recordings rendered their clinical usefulness unclear.46,47,48 Recently, Sasaki et al reported three technical ameliorations of the technique in order to improve the reliability of the method: the introduction of a new light-stimulating device to guarantee retinal stimulation even when the scalp is reflected; the use of electroretinography in order to avoid VEPs false positive related to technical problem (light-stimulating device displacement) or preexisting visual dysfunction; and the use of TIVA to avoid the effects of inhalation anesthesia.49 These authors reported the application of this technique in five AVMs surgeries (three temporal, one parietal, and one occipital AVMs) out of a total of 100 patients (200 eyes); the criterion for amplitude changes was defined as 50% decrease or increase compared with the control level. The VEP amplitude decreased without recovery to 50% of the control level in 14 eyes and all of these developed postoperative deficits; this group comprehended a patient treated for an occipital AVM who developed hemianopia after surgery. Of 169 eyes without VEP changes in amplitude during surgery, two eyes showed a slight visual defect in both eyes after surgery. San-Juan et al reported a case of occipital AVM surgically resected using cortical VEP monitoring.50 The authors used an intracranial electrode grid (5 x 4) placed over the occipital lobe and, initially, over the AVM to register flash cortical VEPs obtained through binocular stimulation (flash frequencies starting from 1 to 5.1 Hz with a total duration of 10 s); the photic driving was registered on channels 1–2 and 2–3 of the ECoG and correlated with the average of the VEPs. The cortical resection was tailored to avoid those areas from which photic driving could be obtained and the patient did not experience postoperative visual defects.
In eloquent AVM surgery, the role of IONM is related not only to its capability to detect impending ischemia but also to help the surgeon in defining the localization of eloquent cortex. In facts, an AVM located in or adjacent to regions such as sensorimotor, language, and visual cortex can result in the translocation of these functions to neighboring cortical areas. Kombos et al reported the case of a left sensorimotor cortex AVM, in which intraoperative mapping showed an unexcitability of the precentral gyrus, while stimulation of the cortex anterior to the primary motor cortex elicited motor responses.51 During surgery, the central sulcus was identified by phase reversal of the SSEPs and the motor cortex was mapped by direct high-frequency (500 Hz) monopolar anodal stimulation. Thus, stimulation of the primary motor cortex induced no motor response, whereas a motor response was elicited only by stimulation of the cortex anterior to the precentral gyrus. There was no postoperative deterioration of motor function.
10.3.2 IONM during Awake Craniotomy for AVM Surgery
The use of awake craniotomy for AVM surgery remains anecdotal. Recently, Gamble et al52 described the use of subcortical mapping during awake resection of four AVMs in the speech area. A complete speech arrest was noted during mapping of the peri-malformation zone in one case; in a second patient, a speech deficit was noted during the resection of the nidus with subcortical mapping. In all four cases, the AVMs were completely removed without permanent postoperative deficits. The patient who presented a speech deficit during subcortical mapping experienced a mild and transient dysphasia that improved in 6 weeks.
Gabarrós et al53 furthermore emphasized the role of cortical mapping for motor and language areas as an invaluable tool in identifying the eloquent cortex, guiding the dissection to the AVM beneath the cortical surface through the identification of safe sulci. In his study, neurophysiological mapping significantly impacted the decision to completely resect the AVM. These authors reported 12 cases of AVMs in eloquent areas, 5 of which were in speech-related areas and 7 in motor areas. The five patients with tumors in speech-related areas were treated with awake craniotomy. Overall, brain mapping influenced the resection in four patients: in two cases, the AVM nidus was too intimately associated with the eloquent cortex to consent safe resection; in the other two cases, the deep extension of the nidus in proximity with the internal capsule and deep supply from lenticulostriate arteries led to halting the dissection. In these cases of incomplete resection, the nidus were left dearterialized and with preserved draining vein, and directed to radiosurgery. Finally, the authors stated that indications for intraoperative mapping include: preoperative imaging suggestive for language/sensorimotor cortex nearby the AVM; larger AVM with high Spetzler-Martin grade; and patient with unruptured AVM without deficits.
10.3.3 Presurgical Functional Imaging as an Alternative to Awake Surgery for Brain AVMs in language and other cognitive-related areas
Overall, preoperative functional imaging is still of much larger use than awake craniotomies in treating AVM located in cognitively eloquent areas. Nowadays, neuroradiological imaging can detect shifts in functional localization with functional magnetic resonance imaging (fMRI), magnetoencephalography (MEG), magnetic source imaging (MSI), positron emission tomography (PET), Wada testing, and, last but not least, transcranial magnetic stimulation (TMS).54,55
However, it should be considered that both fMRI and PET provide preoperative functional data that are essentially based on metabolic information. This does not necessarily correlate with the neurophysiological information offered by IONM, and vascular cases are typically those where a discrepancy between preoperative fMRI and intraoperative neurophysiological mapping may occur.56 Moreover, as suggested by Ozdoba et al,57 flow abnormalities such as those detected in “high-flow malformations” make it no longer possible to use a task-related increase of blood flow (related to the blood oxygen level dependent—BOLD—response in fMRI), given the reserve capacity of adjacent vessels might already be exhausted.
The reliability of preoperative functional neuroimaging is certainly higher for the localization of motor rather than cognitive, and especially language-related, areas. Lehericy et al demonstrated that fMRI incorrectly quantified contralateral language reorganization in AVMs with significant flow abnormalities.58 Thus, although preoperative functional imaging serves as a screening test for major reorganization of the eloquent cortex, intraoperative neurophysiology remains the gold standard in the identification of highly eloquent cortex, such as sensorimotor and language areas. Cannestra et al,59 for example, showed that in spite of fMRI findings, suggesting feasible resection for central region AVMs, in two cases intraoperative cortical stimulation showed eloquent cortex involving the nidus, thus halting the resection.
Navigated TMS is a promising technique that has already showed great reliability in the presurgical planning of brain tumor surgery. Its role in surgery of brain AVMs remains undetermined but preliminary results are encouraging.55
Finally, the interest for tractography is increasing also in AVM surgery, following the much larger experience in brain tumor surgery. Although this technique does not provide any functional information but only an anatomical information on the displacement and orientation of white matter tracts, its role in AVM surgery near the CSTs has been documented by some preliminary reports.60,61
10.4 IONM during Endovascular Procedures
In the comprehensive management of cerebral AVMs, the development and refinement of endovascular techniques has progressively changed the treatment algorithm and their utilization prior to surgical resection has been widely accepted, especially in high-grade Spetzler-Martin AVMs.62,63,64 However, the risk and benefits of this modality has to be incorporated into the overall risk profile for the treatment of AVMs.65 In case of an AVM in eloquent area, a provocative test is a useful tool that enables the surgeon to distinguish between AVM direct feeders and normal brain arteries. A provocative test is based on the superselective injection of sodium amytal—a short-acting barbiturate—or similar in the AVM feeders followed by a clinical examination of the patient. If a new neurological deficit appears, the test is positive and it means that the vessel injected has a functional role and cannot be safely embolized. Rauch et al showed that the amytal test is a good predictor of neurological sequelae after embolization, even if superselective angiogram does not show supply of the normal parenchymal from the vessel(s) tested.66 The authors reviewed their experience in 147 AVM embolization procedures for which 30 patients were submitted to preoperative amytal test with EEG monitoring and clinical examination. There were 23 positive results: 18 of them did not undergo embolization, whereas 5 patients did. Among these five patients, two (40%) developed postoperative deficits. However, the authors underlined that many patients developed EEG changes in absence of clinical symptoms. In fact, in a different article by the same group, out of a total 109 amytal test, 23 produced positive results; however, only 12 positive results were detected by clinical examination, which means that almost one-half were missed without EEG monitoring.67 Furthermore, Paiva et al confirmed the relationship between focal or diffuse low-frequency EEG abnormalities and poor clinical outcome in patients submitted to cyanoacrylate AVM embolization.68 In order to improve the utility and efficacy of the provocative test, Paulsen et al reported their experience during the treatment of 17 patients with rolandic AVMs.69 In this series, in 16 of 17 patients, SSEPs were also registered in order to augment the physical examination. A total of 23 embolizations were performed in 17 patients. In two patients, the embolization was aborted based on the positive amytal test: the first patient showed a greater than 50% amplitude reduction of the cortical SSEP and physical examination showed a transient new neurological deficit; the second one was treated under general anesthesia (pediatric patient) and the result of the provocative test was the significant amplitude reduction of the cortical SSEPs. There were no permanent postoperative deficits and four minor transient deficits. Moo et al proposed an evaluation of the provocative test based on neurologic and cognitive testing customized to AVM size and location.70 Out of a total of 29 provocative test, 27 yielded no clinical deficits, whereas in 2 cases the amytal injection caused cognitive deficits that were neuroanatomically attributable to the eloquent cortex in the region of the AVM. In these two patients, the embolization was aborted and the patients did not experience new postprocedure cognitive deficits.
Provocative tests can also be applied to the evaluation of visual fields during embolization of occipital AMVs. Tawk et al reported their experience on 13 patients with occipital AVMs who underwent 39 Wada test before embolization.71 Patients were treated under conscious sedation. Provocative test induced neurological deficit in six patients; the positive result of the test led the authors to abort the procedure in four cases, whereas the advancement of the catheter tip more distally allowed them to proceed with embolization in the remaining two patients. Despite passing the provocative test, one patient developed a permanent visual deficit few hours after the endovascular procedure. No other patient presented alteration of the visual field after the procedure.
Endovascular management of AVMs may often require general anesthesia, either because the patient is not collaborative (e.g., pediatric patients) or because of operator preference. In such a situation, clinical examination is not possible and the only way to assess functional integrity of the sensory and motor pathways is IONM unless a wake-up test is performed. Under these circumstances, the combination of provocative tests and IONM can be used to predict the effect of embolization (► Fig. 10.3). Besides the use of Amytal, which selectively blocks the gray matter, a local anesthetic such as lidocaine is used to selectively blocks the white matter.72 Thus, the combination of these two provocative agents allows testing both neuronal and axonal transmission, and its use has been well documented during endovascular procedures for spinal cord AVMs, while there is limited experience for brain AVMs.73 A positive provocative test is usually considered as a 50% decrease of SSEP amplitude and/or MEP disappearance. A positive test indicates that the vessel distal to the microcatheter is functionally relevant and cannot be embolized. Whenever a provocative test is positive, embolization is not performed from that specific catheter position. Instead, superselective catheterization or embolization through a different feeder are preferred. If the test is negative (no IONM changes), embolization can proceed. This strategy (evoked potentials and provocative tests) has proved to be very sensitive: almost invariably, patients with negative provocative tests followed by embolization have a good outcome without new neurological deficits.74 Unfortunately, the specificity of this technique has not been tested given that embolization is considered too risky in case of positive provocative tests.
Fig. 10.3 Pharmacological provocative test (PT) during endovascular embolization for brain AVMs. A schematic illustration of a brain AVM is presented (left-hand panels) together with the result of the provocative test (right panels). Negative PT. Left-hand upper panel: The microcatheter (in yellow) is advanced into a feeder of the AVM nidus. Before the embolization, a pharmacological provocative test with either amytal and/or lidocaine is performed. If the microcatheter is selectively advanced into an artery feeding only the nidus and not eloquent cortex or subcortical white matter tracts, the drug (green dots) will diffuse only into the AVM and no changes in the evoked potentials (either SSEPs or MEPs) will occur. Accordingly, mMEPs are unchanged (right-hand upper panel). Positive PT. Left-hand lower panel: The microcatheter (in yellow) is not superselectively advanced into a feeder of the AVM nidus. In this situation, the drug (green dots) will diffuse not only into the AVM but also to either eloquent cortex or subcortical white matter tracts. Accordingly, either SSEPs and/or MEPs changes will occur (right lower panel).
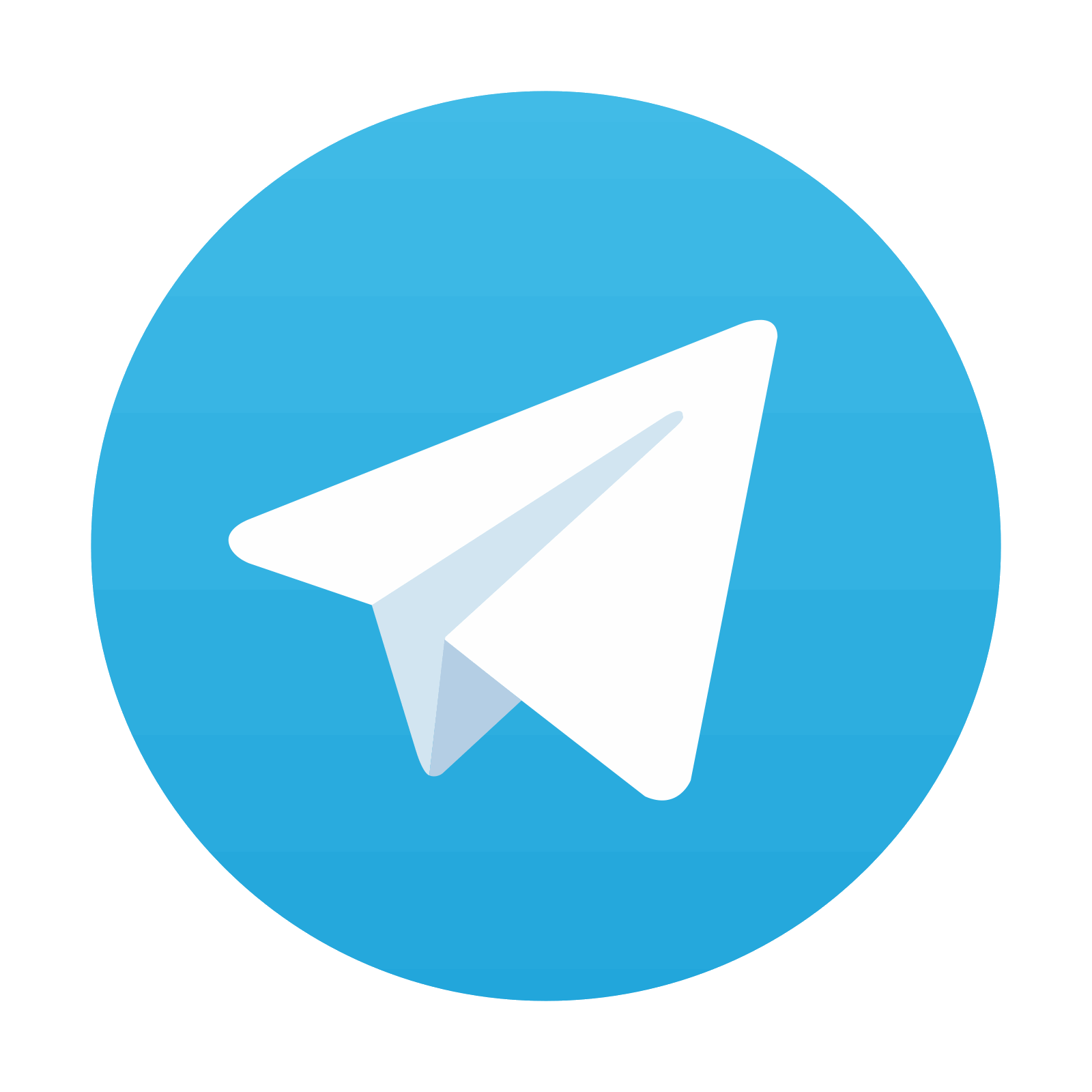
Stay updated, free articles. Join our Telegram channel
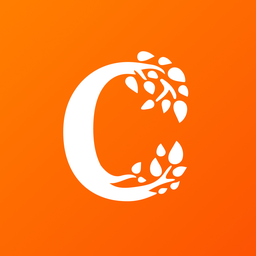
Full access? Get Clinical Tree
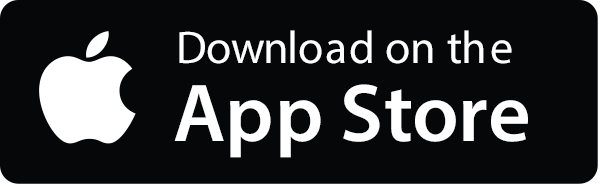
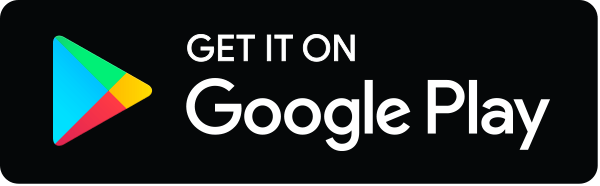