Surgical excision of brain tumors provides a means of cytoreduction and diagnosis while minimizing neurologic deficit and improving overall survival. Despite advances in functional and three-dimensional stereotactic navigation and intraoperative MRI, delineating tissue in real time with physiologic confirmation is challenging. Raman spectroscopy has potential to be an important modality in the intraoperative evaluation of tissue during surgical resection. In vitro experimental studies have shown that this technique can be used to differentiate normal brain tissue from tissue with infiltrating cancer cells and dense cancerous masses with high specificity, indicating the feasibility of this method for in vivo application.
Key points
- •
Surgical excision of brain tumors provides a means of cytoreduction and diagnosis while minimizing neurologic deficit and improving overall survival.
- •
Raman spectroscopy is a promising investigative and diagnostic tool for neurosurgery, and provides rapid, nondestructive molecular characterization in vivo or in vitro for biopsy, margin assessment, or laboratory uses.
- •
A review is presented of Raman spectroscopy techniques for clinical practice, particularly in distinguishing between normal and diseased tissue without the assistance of a pathologist, or to augment pathologic findings.
Introduction
Raman spectroscopy has been identified as a promising investigative and diagnostic tool in neurosurgery because it provides rapid, nondestructive molecular characterization in vivo or in vitro for biopsy, margin assessment, or laboratory use. Spectroscopic information resulting from Raman active functional groups of nucleic acids, proteins, lipids, and carbohydrates in tissue allows evaluation and characterization. This article highlights the need for such an instrument, summarizes neurosurgical Raman work to date, and discusses what the future of neurosurgical Raman spectroscopy might look like.
The estimated incidence of new primary brain and central nervous system (CNS) tumors for 2016 is 77,670 with 16,616 predicted deaths resulting from malignant tumors. With advances in intraoperative imaging, surgical excision is becoming more relevant for brain tumors, providing a means of cytoreduction and diagnosis while minimizing neurologic deficit and improving overall survival. Even in primary malignant tumors such as high-grade gliomas, for which prognosis is poor, the extent of resection is associated with improved mortality and outcomes. Glioblastoma multiforme (GBM), an extremely aggressive primary brain tumor with an average life expectancy of approximately 12 to 18 months, accounts for 15.4% of all CNS and primary brain tumors. A recent study reviewed tumor volumetrics in 500 newly diagnosed patients with supratentorial GBM and found a statistically significant difference in survival with subtotal resections as low as 78% for survival benefit. This work, among growing literature, shows that early maximal resection while preserving a patient’s functional status is critical for optimal patient outcome, and this suggests a need for new technologies designed to achieve maximal safe resections.
Many tools have been developed to aid neurosurgeons with intraoperative tumor identification and delineation. Image-guided surgical techniques include intraoperative MRI, fluorescence-guided surgery, neuronavigation, and ultrasonography. As an example, fluorescence-based imaging methodologies that rely on endogenous autofluorescence are briefly highlighted. Autofluorescence is a well-known phenomenon in which a biological substrate is excited with a suitable wavelength of light. The emission of light in the ultraviolet (UV), visible and near-infrared (NIR) range results from the presence of fluorophores that naturally occur in tissues such as proteins (elastin and collagen), flavins, vitamin A, and fatty acids. However, steady state measurements that rely solely on fluorescence emission intensity are affected by numerous factors. These factors include irregular tissue surfaces, nonuniform sample illumination, and the presence of endogenous absorbers in the biological substrate. Fluorescence lifetime imaging microscopy (FLIM) resolves some of the difficulties in quantifying fluorescence intensities. FLIM produces spatially resolved images of fluorophore lifetime. The technique involves exciting the sample with repetitive light pulses and observing the fluorescence response. Sun and colleagues performed a pilot study using an endoscopic fluorescence lifetime imaging for intraoperative identification of GBM and showed the contrast between normal and tumor tissue. Time-resolved fluorescence spectroscopy (TR-LIFS) is also used to extract fluorophore lifetime but, instead of image acquisition, the method records the time-resolved spectrum at a single point. Butte and colleagues used TR-LIFS to detect the fluorescence lifetime differences between normal brain tissue and glioma in vivo. Both time-resolved fluorescence spectroscopy and imaging techniques have complex instrumental setups making the translation to a clinical setting difficult. In addition, because of the presence of multiple fluorescence molecules, the measured signal from the tissue is a distribution of fluorescence decays, making the methods susceptible to background interference such as blood. Also, there is no clear-cut interpretation of the data in terms of individual components.
Bridging the gap between maintaining neurologic function and comprehensive tumor cell removal is critical. Direct histologic evaluation through techniques such as mass spectrometry is gaining momentum but many techniques are severely limited by their time-based nature. Raman spectroscopy has potential to be an important modality for intraoperative evaluation of tissue during surgical resection. It is a reagentless, nondestructive optical technique with demonstrated ability to detect changes in the chemical composition and/or molecular structure between diseased and healthy tissue. Although most Raman spectroscopy studies are exploratory, in vitro experimental studies have shown that this technique can be used to differentiate normal brain tissue from tissue with infiltrating cancer cells and dense cancerous masses with high specificity. Further, this technique has potential to be used in conjunction with imaging modalities such as MRI and ultrasonography for more in-depth intraoperative characterization of residual tumor. This article provides contemporary Raman spectroscopy data and further understanding of the Raman effect on biological tissues.
Introduction
Raman spectroscopy has been identified as a promising investigative and diagnostic tool in neurosurgery because it provides rapid, nondestructive molecular characterization in vivo or in vitro for biopsy, margin assessment, or laboratory use. Spectroscopic information resulting from Raman active functional groups of nucleic acids, proteins, lipids, and carbohydrates in tissue allows evaluation and characterization. This article highlights the need for such an instrument, summarizes neurosurgical Raman work to date, and discusses what the future of neurosurgical Raman spectroscopy might look like.
The estimated incidence of new primary brain and central nervous system (CNS) tumors for 2016 is 77,670 with 16,616 predicted deaths resulting from malignant tumors. With advances in intraoperative imaging, surgical excision is becoming more relevant for brain tumors, providing a means of cytoreduction and diagnosis while minimizing neurologic deficit and improving overall survival. Even in primary malignant tumors such as high-grade gliomas, for which prognosis is poor, the extent of resection is associated with improved mortality and outcomes. Glioblastoma multiforme (GBM), an extremely aggressive primary brain tumor with an average life expectancy of approximately 12 to 18 months, accounts for 15.4% of all CNS and primary brain tumors. A recent study reviewed tumor volumetrics in 500 newly diagnosed patients with supratentorial GBM and found a statistically significant difference in survival with subtotal resections as low as 78% for survival benefit. This work, among growing literature, shows that early maximal resection while preserving a patient’s functional status is critical for optimal patient outcome, and this suggests a need for new technologies designed to achieve maximal safe resections.
Many tools have been developed to aid neurosurgeons with intraoperative tumor identification and delineation. Image-guided surgical techniques include intraoperative MRI, fluorescence-guided surgery, neuronavigation, and ultrasonography. As an example, fluorescence-based imaging methodologies that rely on endogenous autofluorescence are briefly highlighted. Autofluorescence is a well-known phenomenon in which a biological substrate is excited with a suitable wavelength of light. The emission of light in the ultraviolet (UV), visible and near-infrared (NIR) range results from the presence of fluorophores that naturally occur in tissues such as proteins (elastin and collagen), flavins, vitamin A, and fatty acids. However, steady state measurements that rely solely on fluorescence emission intensity are affected by numerous factors. These factors include irregular tissue surfaces, nonuniform sample illumination, and the presence of endogenous absorbers in the biological substrate. Fluorescence lifetime imaging microscopy (FLIM) resolves some of the difficulties in quantifying fluorescence intensities. FLIM produces spatially resolved images of fluorophore lifetime. The technique involves exciting the sample with repetitive light pulses and observing the fluorescence response. Sun and colleagues performed a pilot study using an endoscopic fluorescence lifetime imaging for intraoperative identification of GBM and showed the contrast between normal and tumor tissue. Time-resolved fluorescence spectroscopy (TR-LIFS) is also used to extract fluorophore lifetime but, instead of image acquisition, the method records the time-resolved spectrum at a single point. Butte and colleagues used TR-LIFS to detect the fluorescence lifetime differences between normal brain tissue and glioma in vivo. Both time-resolved fluorescence spectroscopy and imaging techniques have complex instrumental setups making the translation to a clinical setting difficult. In addition, because of the presence of multiple fluorescence molecules, the measured signal from the tissue is a distribution of fluorescence decays, making the methods susceptible to background interference such as blood. Also, there is no clear-cut interpretation of the data in terms of individual components.
Bridging the gap between maintaining neurologic function and comprehensive tumor cell removal is critical. Direct histologic evaluation through techniques such as mass spectrometry is gaining momentum but many techniques are severely limited by their time-based nature. Raman spectroscopy has potential to be an important modality for intraoperative evaluation of tissue during surgical resection. It is a reagentless, nondestructive optical technique with demonstrated ability to detect changes in the chemical composition and/or molecular structure between diseased and healthy tissue. Although most Raman spectroscopy studies are exploratory, in vitro experimental studies have shown that this technique can be used to differentiate normal brain tissue from tissue with infiltrating cancer cells and dense cancerous masses with high specificity. Further, this technique has potential to be used in conjunction with imaging modalities such as MRI and ultrasonography for more in-depth intraoperative characterization of residual tumor. This article provides contemporary Raman spectroscopy data and further understanding of the Raman effect on biological tissues.
Raman spectroscopy
Interest in clinical spectroscopy is increasing because of the potential of vibrational spectroscopic techniques for noninvasive tissue diagnosis. Raman spectroscopy and infrared (IR) spectroscopy probe molecular vibrations associated with chemical bonds in a sample to obtain information on molecular structure, composition, and intermolecular interactions.
The Raman effect was discovered in 1928 by C.V. Raman when he observed that light traveling through various liquids scatters differently, in a behavior distinct from fluorescence. The basic process of scattering is the absorption of and reemission of electromagnetic radiation by molecules. The mechanism associated with scattering is induced electric dipole radiation. Light incident on matter can scatter at the same optical frequency as the incident radiation (ie, the induced dipole moment in a molecule oscillates and radiates at the same frequency as the incident light). This process is termed elastic scattering (Rayleigh scattering). Light can also scatter at frequencies that differ from the original radiation. This process is called inelastic scattering and, unlike the elastic process, it involves an energy transfer between the incident photons and a material. An inelastic process, termed the Raman effect, occurs in approximately 1 in 10 7 photon interactions with matter and results from modulations in the induced dipole moment within molecules. Changes in polarizability with molecular vibration cause the induced dipole moment to oscillate at frequencies other than the incident light. Radiation emitted at longer wavelengths (downshifted frequencies) than the incident light is termed Stokes scattering, which occurs when a molecule is promoted from the ground state to a virtual energy level (an intermediate electron state with energy lower than a real electronic transition) then relaxes to a vibrational state. Radiation emitted at shorter wavelengths (upshifted frequencies) than the original radiation is known as anti-Stokes scattering. This process occurs when a molecule is initially in a vibrational state and relaxes to the ground state after scattering.
With conventional Raman spectroscopy, the effect is independent of wavelength because no real energy states are involved (only virtual states), and this is termed nonresonance Raman. However, certain substances when exposed to electromagnetic radiation can produce a strong fluorescence signal that overlaps the Raman signal. Raman scattering and fluorescence are competing phenomena that have a similar origin. The Raman effect corresponds with the absorption and subsequent emission of a photon via an intermediate electron state, as shown in an energy level diagram ( Fig. 1 ). Molecules are excited to a virtual energy level for a short period, on the order of picoseconds, before an emitted photon results; whereas in fluorescence the incident light is absorbed by a molecule and reemitted from electronically excited states after a resonance time on the order of nanoseconds.
In contrast, resonance Raman spectroscopy, a variant of conventional Raman, measures molecular vibrations in a wavelength-dependent manner. When the wavelength of the exciting source coincides with an electronic transition of the molecule a resonance effect is observed and the intensity of some Raman active vibrations can be increased by a factor of 10 2 to 10 6 .
There are 4 primary components in a conventional Raman spectroscopy system: (1) the light source (usually a laser), (2) the spectrometer, (3) a filter to block the laser line and allow Raman scatter to pass, and (4) a detector. Fig. 2 shows a basic Raman spectrometer. The recorded inelastic scattering results in a Raman spectrum or fingerprint, which can be used in imaging and diagnostics. The x-axis corresponds with the change in energy of the scattered photon; the y-axis corresponds with the photon count. Energy shifts correlate to types of bonds, such as C=H, C-C, or CH 2 twist, based on quantized energy levels of each molecular bond. The amount, location, and intensity of peaks vary based on the number of vibrational bonds in a molecule and their ensemble interactions with each other. Biological spectra can be complex. For example, l -phenylalanine (C 6 H 5 CH 2 CHCOOH), an important tumor marker, shows 63 peaks in the Raman fingerprint ( Fig. 3 ).
When using Raman with large molecules, the group contribution approach is used whereby the largest peaks come from the bonds that occur most frequently. The distinctive peak for l -phenylalanine between 1000 and 1008 cm −1 results from C-C bonds and ring breathing modes of benzene, which are the most prevalent bond vibrations. Some molecular peaks may not be apparent in the spectrum depending on bond strength and shape. If the bond does not change its polarizability, it is not easily detectable. For example, water has a weak Raman signal because of its symmetric polarity. In tissue diagnostics, this can be advantageous. In contrast, infrared spectroscopy signals can be overwhelmed by water emissions.
The Raman spectrum of tissue induces many peaks representative of the plethora of cellular constituents (see Fig. 3 ). Distinct differences between pathologic conditions are shown as different intensities of the same peak or peak shifting based on binding conformation. Occasionally, there is a unique peak from a different moiety, such as calcifications or hemorrhage. Biological tissues are largely made up of the same molecules, but the quantity and interactions of the molecules vary between tissue types. For example, tumors use glycolysis to generate ATP, which produces more pyruvate, which converts to lactate and subsequently to the amino acid alanine, resulting in stronger protein peaks.
Early applications of neurologic Raman spectroscopy
In 1990, Raman spectroscopy was first applied to characterize parenchymal water content in situ. Normal and edematous brain tissue showed similar peak intensities attributed to protein CH bonds. Although water produces a weak Raman signal, when coupled with the OH water peak, the OH/CH ratio gives a relative comparison of water content.
Later, Mizuno and colleagues used Raman spectroscopy to differentiate gray from white matter in a rat model by examining the spectral ratio of protein to lipid content. In this case, gray matter has an abundance of proteins, whereas white matter has increased phospholipid levels because of its myelination. Numerous studies have contributed a wealth of information to the molecular fingerprints of biological tissues, and several Raman peak assignment tables are available. Table 1 provides a summary of peak information for brain diagnostics.
Raman Peak (cm −1 ) | Assignment | Citation |
---|---|---|
427–430 | Cholesterol/cholesterol ester | |
Higher in white matter than gray matter | ||
Cholesterol deposits found in tumor sections | ||
429 | Bending PO 4 in hydroxyapatite, deposits found in tumor tissues | |
457 | Melanin, found in metastatic melanoma | |
467–475 | Glycogen and polysaccharides seen in PA and MG with SERS | |
482 | Glycogen, seen in metastatic renal cell carcinoma | |
491 | Cholesterol | |
498–500 | Nucleic acids/nucleotides | |
525 | S-S stretch mode of gauche-gauche-trans form, higher in synaptosomal fraction than myelin | |
538 | Cholesterol ester, cholesterol deposits found in tumor sections | |
544–548 | Cholesterol | |
Higher in white matter than gray matter | ||
553 | S-S trans-gauche-trans mode, higher in synaptosomal fraction than myelin | |
558–566 | Tryptophan, higher in GBM, ODG tumor when measured with SERS | |
583 | Bending of PO 4 in hydroxyapatite, deposits found in tumor tissues | |
597–599 | Melanin, seen in metastatic melanoma | |
607–608 | 607: glycerol | |
608: cholesterol | ||
Higher in white matter than gray matter | ||
Cholesterol deposits found in tumor sections | ||
613–622 | Cholesterol ester | |
Cholesterol deposits found in tumor sections | ||
622/624: phenylalanine (protein) associated with necrosis | ||
Present in SERS, higher in ODG tumor | ||
642–645 | Tyr (protein) associated with necrosis | |
648–655 | C-C and C-S stretch of protein (SERS) | |
Ratio of 724:655 is significantly different between tumor/normal | ||
661–669 | 666: Heme associated with hemorrhage | |
667, 669: nucleic acid/nucleotide associated with necrosis, tumor | ||
680–683 | Nucleic acids | |
700, 703/704 | C-N stretch mode from choline head in phosphatidylcholine and sphingomyelin | |
720:701 ratio increases in GBM | ||
Cholesterol | ||
Variable in normal tissue | ||
Higher in white matter than gray matter | ||
Distinguishes myelin fraction from synaptosomal | ||
Deposits found in tumor sections | ||
Cholesterol content decreases from corpus callosum to cortex | ||
Decreased cholesterol/phospholipid content in tumor compared with normal | ||
C-S stretch from methionine, cysteine, and cysteine residues of proteins (less likely) | ||
716–719 | Choline in head group of sphingomyelin and phosphatidylcholine, phosphatidylethanolamine | |
Higher in normal than tumor | ||
720:701 ratio increases in GBM | ||
Stronger in GBM than MG | ||
722–728 | DNA, RNA base ring breathing mode, C-S of proteins, CH 2 rocking of adenine, NADH, FADH (SERS), nucleotides | |
Ratio of 724:655 signifies difference between tumor/normal | ||
Seen in cell nuclei | ||
Increased DNA/RNA in tumor with respect to normal | ||
727 | Melanin, seen in metastatic melanoma | |
739–759 | Heme (blood) | |
746 | Phosphorus-oxygen-phosphorus bonds symmetric stretch of phospholipids (predominant in white matter and myelin fraction) | |
749–751 | Nucleotides | |
757–760 | Tryptophan | |
Associated with necrosis | ||
781–795 | O-P-O stretch of DNA/nucleic acids, seen in cell nuclei | |
Associated with tumor | ||
Nucleic acid band with the least overlap with lipid, cholesterol, and protein, making it a strong marker for mitosis index | ||
800 | Quartz substrate (removed via processing) | |
826–830 | Tyr, proline | |
830 | Phosphate backbone of β-DNA conformation | |
Higher in tumor, necrosis than in normal | ||
845 | Cholesterol | |
851–852 | Tyr, associated with necrosis | |
850–855 | Glycogen, seen in metastatic renal cell carcinoma and high-grade tumors | |
856 | Polysaccharides | |
Collagen | ||
Strong in glioma | ||
Seen in dura mater but not meningioma | ||
865 | Phosphatidylethanolamine, lower in tumor than normal | |
867 | Glycogen, seen in metastatic renal cell carcinoma | |
873 | Phosphatidylcholine | |
875–878 | Tyr, higher in astrocytoma cells than astrocyte cells | |
Choline group in PC and sphingomyelin | ||
905–908 | Glucose level, increased in PA, as measured by SERS | |
925–926 | C-C in peptide backbone | |
Cholesterol | ||
935–939 | C-C of peptide backbone, collagen | |
Seen in dura mater but not meningioma | ||
941 | Glycogen, seen in metastatic renal cell carcinoma | |
956 | Carotenoid (present in schwannoma, not present in normal tissue) | |
C-C vibration of protein, carotenoids, hydroxyapatite, collagen | ||
Increased in lung metastases tumor, GBM, ODG, PA, EP, MG as measured by SERS | ||
958–960 | Stretching vibrations of PO 4 in hydroxyapatite, deposits found in neurocytoma, metastatic melanoma, psammoma body of meningioma | |
Stronger in necrosis than tumor | ||
961 | Higher cholesterol content in white matter than gray matter | |
969–977 | Tricalcium phosphate Ca 3 (PO 4 ) calcification seen in schwannoma, necrosis | |
976 | Melanin, seen in metastatic melanoma | |
985 | Calcification | |
990 | Phosphate ions (in PBS) | |
1002–1006 | Ring breathing mode of phenylalanine of protein, heme | |
Higher in lung met tumor, GBM, EP (SERS), astrocytoma, necrosis than in normal | ||
Stronger in necrosis than tumor | ||
Increased in high-grade tumors | ||
Slightly increased after radiation exposure | ||
Weaker after brain injury | ||
Carotenoid (present in schwannoma, not present in normal tissue) | ||
Heme/hemorrhage | ||
1032 | C-H of phenylalanine | |
Increased in necrosis | ||
Weaker after tissue fixation | ||
Higher in glioma tissue and cells than normal tissue and astrocyte cells | ||
1042–1049 | C-O and C-N stretch of protein, higher in lung met tumor, MG measured by SERS | |
1050 | Quartz substrate (removed via processing) | |
1060–1066 | C-O stretch and C-O-C symmetric stretch, C-C stretch of phospholipids (side chains specifically) and cholesterol | |
Strongest in white matter and myelin fraction | ||
Strong in normal, weaker in tumor/necrosis | ||
Higher in Alzheimer hippocampus than normal hippocampus | ||
Stronger in necrosis than tumor | ||
Cholesterol deposits found in tumor sections | ||
1071 | Stretching vibration of PO 4 in hydroxyapatite, deposits found in tumors | |
1080–1090 | C-C stretch and PO 2− symmetric stretching of phospholipids and nucleic acids | |
C=O vibration of ester/aldehyde | ||
Higher in gray matter than white matter | ||
Higher in white matter than gray matter and tumor | ||
Seen in metastatic tumors | ||
Seen in cell nuclei | ||
Stronger in necrosis than tumor | ||
Stronger in gray matter than tumor | ||
1088–1097 | C-N stretch of protein, higher in GBM, ODG, PA, EP, MG when measured by SERS | |
1088 | Lipid/glycogen | |
Seen in metastatic renal cell carcinoma, high-grade tumors | ||
Higher in Alzheimer hippocampus than normal hippocampus | ||
1094–1100 | Phosphate backbone of β-DNA conformation | |
Marker for mitotic figure | ||
Higher in glioma tissue and cells than normal tissue and astrocyte cells | ||
1122 | Glycogen, seen in metastatic renal cell carcinoma | |
1124 | Melanin, seen in metastatic melanoma | |
Heme/hemorrhage | ||
1126–1133 | C-C stretch of phospholipids (side chains), cholesterol | |
C-C and C-N stretch of protein/glucose | ||
CH2 vibration | ||
Stronger in white matter and myelin fraction | ||
Stronger in glioma, suggesting higher concentration of trans conformation hydrocarbon chains in tumor lipids | ||
Stronger cholesterol/phospholipid content in normal than tumor | ||
Stronger in Alzheimer hippocampus than normal hippocampus | ||
Stronger in necrosis than tumor | ||
Cholesterol deposits found in tumor sections | ||
1142 | Lipid | |
1157–1159 | Carotenoid, present in schwannoma and some GBMs, not present in normal tissue | |
Stronger in necrosis than tumor | ||
1174–1175 | Protein, higher in lung met tumor (measured by SERS), and in GBM than normal | |
Cholesterol/lipid band appearing after brain injury | ||
1206–1208 | Amide III, higher in ODG, MG as measured by SERS | |
Phenylalanine | ||
Highest in necrosis, lowest in normal, tumor intermediate | ||
1212 | Oxygenated hemoglobin | |
1228 | Cholesterol/lipid band appearing after brain injury | |
Seen in lung carcinoma met | ||
1225–1300 | Amide III band (collagen/protein, amino acids) | |
Mainly α-helix conformation in normal brain | ||
Shifts to lower wavenumber in glioma, suggesting random coil structure | ||
Intensity and position are variable in normal brain | ||
Higher in lung met tumor, GBM, ODG, PA, astrocytoma cells | ||
Associated with necrosis | ||
Shoulder in necrosis, weaker shoulder in tumor | ||
Higher in glioma tissue and cells than normal tissue and astrocyte cells | ||
1231–1258 | Heme (blood) | |
1260 | Overlaps with unsaturated fatty acids/phospholipids | |
Lipids higher in dura than meningioma | ||
1268–1270 | δ=CH unsaturated fatty acids | |
Diagnostically significant for normal tumor and tumor grade | ||
Decreases with increasing saturation | ||
Higher in gray matter than white matter | ||
Higher in white matter than gray matter | ||
Slightly increased after radiation exposure | ||
1296–1302 | CH 2 twist and wag of phospholipids, fatty acid, cholesterol | |
Not a diagnostically significant/variable intensity/position | ||
Increases with increasing saturation | ||
Stronger in white matter and myelin fraction than gray matter and tumor | ||
Seen in metastatic tumors, astrocytoma cells | ||
Higher in Alzheimer hippocampus than normal hippocampus | ||
Stronger in necrosis than tumor | ||
Cholesterol deposits found in tumor sections | ||
1302–1305 | Lipid/glycogen (renal cell carcinoma) | |
Cholesterol ester | ||
1313 | CH 3 /CH 2 deformation of lipids and proteins | |
Strongest in necrosis, intermediate in tumor, weakest in normal | ||
1320–1330 | C-H deformation or CH 2 bend (protein) increased in lung met tumor, GBM, ODG, EP, MG (SERS) | |
1333–1343 | Aliphatic amino acids, including tryptophan, nucleic acids | |
Glycogen | ||
Associated with necrosis | ||
Increased in high-grade tumors and metastatic renal cell carcinoma | ||
1346 | Heme/hemoglobin | |
CH deformation of lipid/protein | ||
1366 | Higher in GBM (SERS) | |
1372–1376 | DNA bases, seen in cell nuclei | |
1397 | CH 2 /CH 3 deformation of lipids and proteins | |
1400–1404 | Melanin, seen in metastatic melanoma | |
Quartz substrate (removed via processing) | ||
1422 | Nucleotides | |
1439–1455 | CH 2 /CH 3 deformation of lipids side chains, proteins, amino acids, cholesterol/cholesterol ester | |
Intensity and position are variable in normal brain | ||
Higher in white matter than gray matter and tumor | ||
Higher in Alzheimer hippocampus than normal hippocampus | ||
Lipids higher in normal than meningioma | ||
Cholesterol deposits found in tumor sections | ||
Higher in ODG, EP, MG renal cell carcinoma met, astrocytoma | ||
Variable in malignant tissues | ||
Associated with necrosis | ||
Higher in glioma cells than astrocytes | ||
1454 | Heme/hemoglobin | |
1460 | Glycogen, seen in metastatic renal cell carcinoma | |
1466 | CH 2 bend of proteins | |
1483–1488 | DNA bases, seen in cell nuclei | |
1518–1527 | Carotenoid | |
Present in schwannoma, some GBMs, not present in normal tissue | ||
Stronger in necrosis than tumor | ||
1540 | Nucleic acid, higher in tumor | |
1550–1555 | Tryptophan | |
Associated with necrosis, slightly weaker in normal, weakest in tumor | ||
1546–1568 | Hemoglobin | |
1575–1588 | C-C stretch of protein, nucleic acids (SERS) | |
Higher in ODG, PA, EP, MG | ||
Seen in cell nuclei, marker for mitosis | ||
Sharp band appears after brain injury | ||
1585 | Heme (blood) | |
1592–1595 | Melanin (metastatic melanoma) | |
1603–1605 | Oxygenated hemoglobin | |
1614 | Aromatic amino acids (protein) | |
1619–1626 | Oxygenated hemoglobin | |
Sharp band appears after brain injury | ||
1635–1640 | Water | |
Glioma has higher water content than normal (but normal tissue may be edematous) | ||
1656–1668 | Amide I band (protein) mainly α-helix in normal brain | |
Also assigned to unsaturated fatty acids (νC=C) | ||
(1645–1675) | Intensity and position are variable in normal brain | |
Associated with necrosis, not as strong in tumor | ||
Higher in normal than tumor | ||
Higher in tumor than normal | ||
Lower unsaturated lipid content as malignancy increases | ||
Lower unsaturation in white matter | ||
Higher in gray matter than white | ||
Higher in white matter than gray matter | ||
Shoulder at 1670 in Alzheimer hippocampus (more deposition of β-amyloid protein?) | ||
Weaker after brain injury | ||
1669–1674 | Steroid ring of cholesterol/cholesterol ester | |
Cholesterol deposits found in tumor sections | ||
1675 | Protein (lung carcinoma met) | |
1684 | Collagen | |
1688 | Higher in EP (SERS) | |
1731–1739 | C=O of ester groups, cholesterol ester | |
Stronger in necrosis than tumor | ||
Lipids higher in dura, cortex than tumor | ||
Deposits found in tumor sections | ||
Not seen in fixed tissues | ||
2850–2853 | CH 2 symmetric stretch of lipid side chain | |
Higher in white matter, lower in gray matter, lowest in tumor | ||
Seen in metastatic renal cell carcinoma | ||
2880–2886 | CH 2 , CH 3 stretch found in lipid (side chain), cholesterol | |
Higher in white matter, lower in gray matter | ||
Lower in tumor | ||
2895 | Glycogen/lipid (metastatic renal cell carcinoma) | |
2929–2938 | CH 2 /CH 3 symmetric stretch of lipid side chains, cholesterol | |
Aliphatic amino acids (associated with necrosis) | ||
2958–2960 | CH 3 asymmetric stretch | |
3010–3015 | Unsaturated lipids, lower in tumor | |
3100–3600 | OH stretch in water | |
3300 | Weak amide band, water | |
Lower in gray matter than white matter |
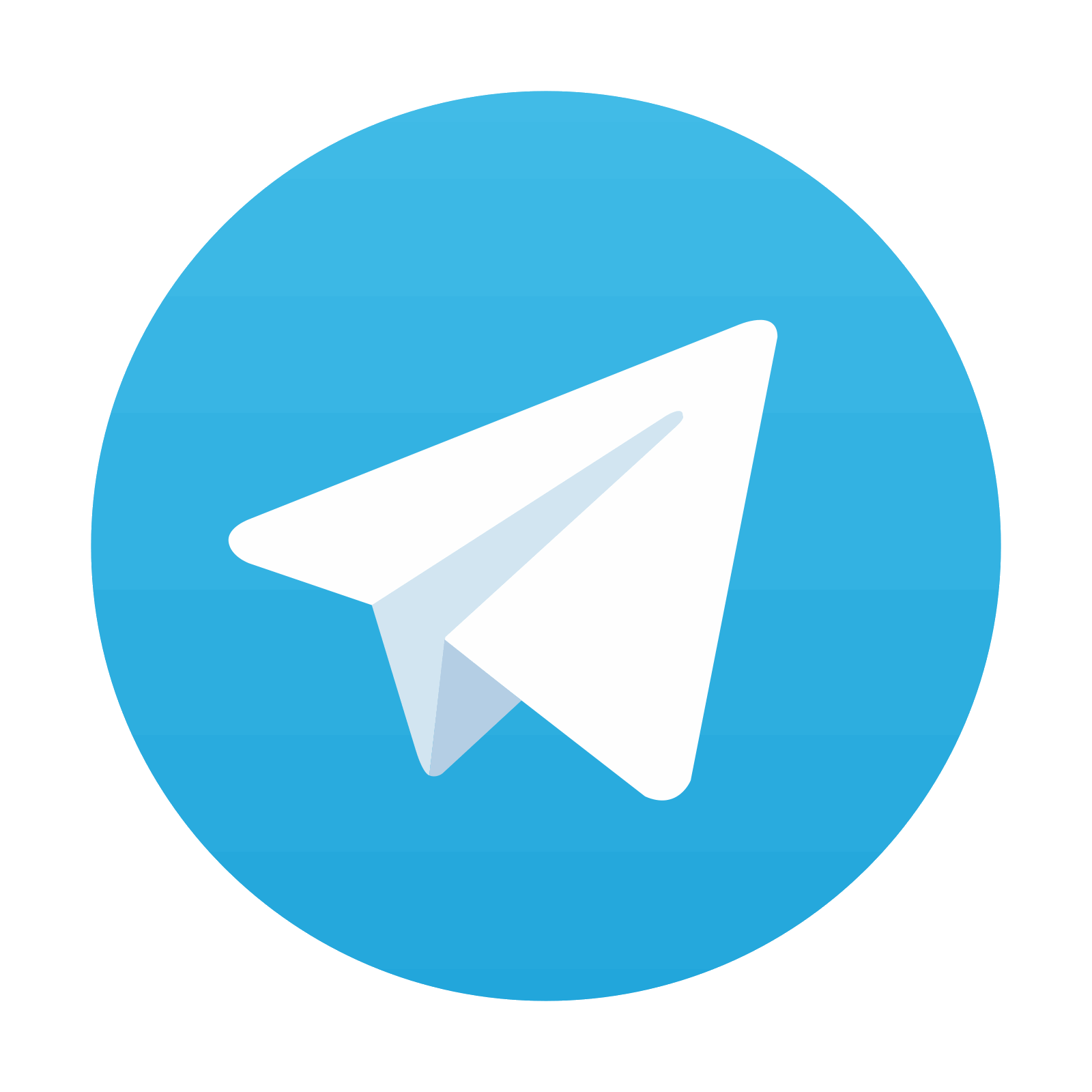
Stay updated, free articles. Join our Telegram channel
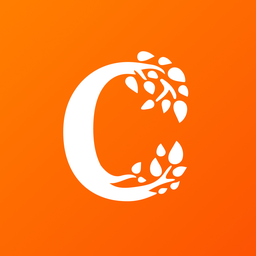
Full access? Get Clinical Tree
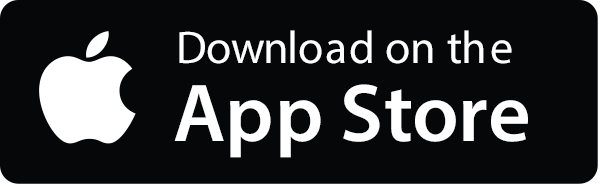
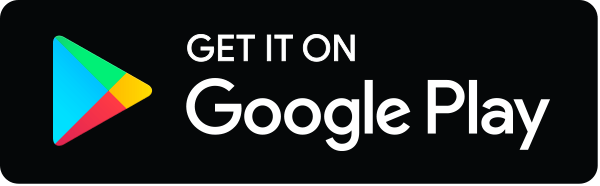
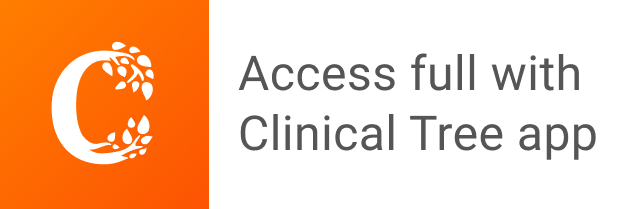