Abstract
Motor neuron diseases, such as amyotrophic lateral sclerosis (ALS) and spinal muscular atrophy (SMA), are devastating neurodegenerative diseases that are progressive and fatal. There is no cure and the current standard of care is merely palliative. For example, riluzole is the only FDA-approved treatment for ALS and extends life for 3–6 months. Due to the inability of conventional therapies to have an impact on these diseases, research in gene and stem cell therapies have come into focus. Gene and stem cell therapies have the potential to make a significant impact on the treatment of ALS and SMA. For this potential to be realized, the optimal gene therapy vector or stem cell needs to be selected. The various options have their pros and cons, which will be discussed in this chapter. It is necessary to take the time to assess and match the disease with the appropriate therapy.
Keywords
Gene therapy, stem cells, viral vectors, delivery routes
Introduction
Motor neuron diseases, such as amyotrophic lateral sclerosis (ALS) and spinal muscular atrophy (SMA), are devastating neurodegenerative diseases that are progressive and fatal. There is no cure and the current standard of care is merely palliative. For example, riluzole is the only FDA-approved treatment for ALS and extends life for 3–6 months. Due to the inability of conventional therapies to have an impact on these diseases, research in gene and stem cell therapies have come into focus.
Gene and stem cell therapies have the potential to make a significant impact on the treatment of ALS and SMA. For this potential to be realized the optimal gene therapy vector or stem cell needs to be selected. The various options have their pros and cons, which will be discussed in this chapter. It is necessary to take the time to assess and match the disease with the appropriate therapy.
Gene Therapy
Gene therapy proposes to replace a defective gene with the correct copy or, to introduce a therapeutic gene that will support and improve the disease environment. Vectors, both viral and nonviral, are used to deliver the therapeutic gene to the target tissue and cells.
Nonviral Vectors
Nonviral vectors consist of a nucleic acid, either naked or complexed with a carrier that aids its passage into the target tissue. They have the advantage of a low risk factor due to the absence of a viral component. Also, there is unlikely to be an immune response caused by the vector, which increases their safety profile. Their capacity for delivering nucleic acid is large and they are easier to synthesize than viral vectors. However, their efficacy is low as they lack the efficient means to reach the nucleus that viral vectors possess. This limits their usefulness as a tool for treating diseases that are systemic or affect more than one organ type. Advances in developing compounds that package the nucleic acid, to prevent degradation and enhance delivery, has resulted in improvements in the effect of nonviral gene therapy. These developments include cationic polymers, some of which have the ability to be retrogradely transported. This would be useful for peripheral neuronal delivery. Some proteins have also been incorporated into nucleic acid carriers due to their ability to bind to cell-surface receptors. For example, tetanus toxin has the ability to target neuronal cells. Fragments of the tetanus toxin have the ability to bind to receptors, be taken up into cells and be transported in a manner similar to the full length toxin. The fusion of tetanus-like peptides with nucleic acid may assist targeting specific cell populations of interest in ALS and SMA. Another option for targeting of nonviral vectors are synthetic nanoparticles. Nanoparticles can be made from a variety of different materials such as lipids and polymers. For example, silica nanoparticles have been demonstrated to result in targeted expression in neuronal cells. This expression was on a par with expression from a herpes simplex virus (HSV) vector and had the advantage of having none of the vector-associated toxicity. It is interesting to note that the majority of the data for nonviral vectors in neuronal cells comes from in vitro studies. Further research is required in vivo to thoroughly evaluate the potential of these nonviral vector options.
Viral Vectors
Viral vectors use the natural ability of viruses to infect host cells and then use the cells’ machinery to express the transgene. They have the genes that allow them to replicate removed from their genome, which renders them safer to use in a gene therapy. Viral vectors can carry either DNA or RNA, can integrate their genetic material into the host genome or exist episomally, and have different tropisms for different tissue and cell types. They also vary in the size of the transgene they can encode. All of these factors have to be taken into account when matching a viral vector with the appropriate disease. The most common viral vectors used include Adenovirus (Ad), Adeno-associated virus (AAV), Lentivirus (LV) and HSV ( Fig. 7.1 ).

Adenovirus
Ad is a nonenveloped, double-stranded DNA virus and has the ability to transduce both dividing and nondividing cells. It most commonly causes respiratory infections. When Ad infects cells, it binds to cell-surface receptors via proteins on its capsid coat. Most Ad serotypes bind to the coxsackie and adenovirus receptor. The virus is internalized by endocytosis prompted by interaction between the viral capsid and integrin receptors on the cell surface. After cell entry, the virus is released from the endosome, interacts with cytoplasmic dynein and microtubules, and is moved toward the nucleus. The Ad capsid docks with the nuclear pore complex protein. The nuclear protein histone H1 protein attaches to the viral capsid and microtubule kinesin-1 disrupts nuclear-pore-complex-docked capsids and the nuclear pore complex. This allows for access of the viral genome to the nucleus. Ad does not integrate into the host genome but exists as a linear episome in the cell nucleus. The transcription and assembly of the progeny virus takes place in the cell nucleus. The progeny virus particles are released from the cell by virus-induced cell lysis.
The development of the Ad vector has gone through several iterations as genes responsible for replication have been deleted from the original viral genome. The first-generation Ad vectors had deletions in the E1 region and/or in the E3 region of the viral genome. The E1 region is responsible for encoding proteins necessary for early gene expression. The E3 region is involved in replication and packaging of the virus. Removal of these genes leaves a transgene capacity of approximately 8 kb. However, as this vector still expressed viral genes, it was found to elicit an immune response and was cytotoxic to cells. This limited the expression of the transgene and, consequently, its usefulness as a vector. The second-generation Ad vectors had more of the viral genome deleted. The E2 and E4 regions were removed along with the original E1 and E3 regions. The deletion of additional regions of the viral genome resulted in a reduced immune response but did not eliminate the problem completely. A side effect of the additional deletions left the vector unable to replicate by itself and it required a helper virus or a stably transfected cell line, expressing the deleted regions, to replicate. The third generation of Ad vectors had most of the viral genome deleted and have also been called gutted Ad vectors. They contain the minimal genes for virus production and packaging and have a capacity of approximately 36 kb.
Ad was first used in clinical trials in cystic fibrosis patients delivering the cystic fibrosis transmembrane receptor gene to the lung epithelium. Subsequent studies demonstrated that the highly immunogenic response elicited by Ad vector administration restricted the duration of transgene expression to approximately 2 weeks. Repeated administration of the Ad vector resulted in reduced expression of the transgene with each additional administration. Advances in Ad vector development toward third-generation Ad vectors have reduced the host immune response but not eliminated it entirely. This has had the effect of extending the expression of the transgene, with expression in nonhuman primate liver detected for up to 2 years. However, to the best of our knowledge, third-generation Ad vectors have not been tested in clinical trials.
The immune response and the resulting limited gene expression have focused use of Ad vectors on cancer treatment. This includes treatment of cancers of the central nervous system (CNS). For cancer treatment, the short-term expression of Ad is an advantage. A number of different strategies have been employed that utilize Ad vectors. They have been used to deliver cytotoxic genes directly to tumors. Another approach has been to deliver a gene that converts a prodrug to its active cytotoxic form. Ad has also been used to express immune-related genes to activate or draw immune cells in the vicinity of the tumor. Another option for Ad vector use is in the area of vaccine development. This has been achieved by raising antigens that recognize the transgene or by inserting the antigen into the capsid. This has been employed to develop vaccines against bioterrorism agents such as anthrax and also against agents such as cocaine.
Ad as viral vector has the capacity to be useful in specific areas such as cancer therapy. However, with its immunogenic profile, it is not a suitable choice for either systemic or organ-targeted gene therapy.
Adeno-Associated Virus
AAV is a small parvovirus virus, approximately 20 nm in size. It is classified as a helper-dependent virus, as it is unable to replicate without coinfection with either an Ad or a Herpes virus. Its genomic material is packaged as a single-stranded DNA molecule. Upon infection, the viral genome is converted to a double-stranded episome with integration into the host genome a rare event. The virion capsid consists of three proteins: VP1, 2, and 3. These proteins are responsible for the binding of the virus to the cell-surface receptors. Different serotypes, or subtypes, of AAV bind to different cell-surface receptors, determining the tropism of each serotype. To package an AAV vector, the transgene cassette must simply be flanked by the inverted terminal repeats (ITRs), which function as an origin of replication for the vector. No viral genes are incorporated into these vectors. AAV2 is the most extensively studied serotype and most AAV vectors use AAV2 ITRs. A limiting factor of AAVs is the cloning capacity of approximately 4.7 kb. Any greater than this and the packaging efficiency of the virus is adversely affected. The first AAV vectors developed were single stranded. The rate-limiting step in using these vectors was second-strand synthesis following cell transduction. This resulted in delayed expression of the transgene. This issue was overcome by the development of double-stranded or self-complementary AAV (scAAV). scAAV has a mutated ITR that is located between two copies of the transgene. The copies of the transgene are complementary to each other and yield a self-annealing double-stranded molecule. This eliminates the need for second-strand synthesis and the transgene is expressed earlier. The disadvantage of scAAV is that it reduces the cloning capacity of the vector to approximately 2.2 kb, limiting the number of genes that can be accommodated in this form of the vector.
As previously stated, AAV2 was one of the first serotypes identified and studied with a view to gene therapy. Since the initial identification of AAV2, a large number of serotypes of AAV have been identified, with each serotype having a different tropism. Along with naturally occurring serotypes, directed evolution has been utilized to develop serotypes with specific tropisms. Directed evolution involves shuffling the capsid genes of naturally occurring AAV to create random chimeras of AAV capsid genes. These are then packaged and screened through either in vitro or in vivo methods. Vectors that transduce target tissues are purified. There may be several rounds of screening to optimize tissue targeting. Other options include engineering the capsid proteins to have a mixture of proteins from two different serotypes to create a mosaic virus or to incorporate targeting proteins into the capsid to direct the tropism of the virus. However, it needs to be borne in mind that engineering capsids can affect the packing of the virus and, consequently, the titer.
Although AAV does not cause disease in humans and does not elicit the immunogenic response of Ad, it does result in the production of anti-AAV antibodies (Abs). These Abs could eliminate the viral vector before the transgene had an effect. This is an important issue for any AAV-mediated therapy that is being translated to the clinic. Suppression of this response with steroids has been sufficient to suppress the loss of the transgene. In addition, Abs can also pose a hurdle at the initiation of treatment, too. A large fraction of the population has already been exposed to naturally occurring AAVs, resulting in the production of neutralizing Abs that can block therapeutic vectors. Currently, the best option to address this problem is to screen prospective patients before they are enrolled in AAV gene therapy trials. Patients with an Ab titer that is too high would not be enrolled. This strategy has been employed in a number of clinical trials, for example the SMA trial that is delivering AAV-9 SMN intravenously (NCT02122952).
AAV serotypes have been demonstrated to be particularly effective in transducing neural tissue. Different serotypes have been tested in several neurodegenerative diseases and have progressed to clinical trials. These include Parkinson’s disease, Alzheimer’s, SMA (NCT02122952), and Canavan’s disease. These trials have repeatedly shown that AAV vectors are safe for clinical use for CNS gene therapies.
Lentiviral Vectors
Lentivirus is a member of the retrovirus family, which also includes HIV-1. They are 80–100 nm in diameter and contain two copies of single-stranded RNA. Following entry into the cell, the RNA is reverse transcribed into linear double-stranded DNA in the cytoplasm. The proviral DNA is then translocated to the nucleus where it stably integrates into the host’s DNA. The integration sites within the host genome are believed to be random within transcriptionally active sites. The viral DNA replicates with the host’s own genome and is passed onto daughter cells. The proviral DNA is then transcribed to produce both the RNA and the viral proteins necessary to produce progeny virus. To acquire its lipid membrane, the progeny lentiviruses bud through the plasma membrane of the host cell.
The first lentiviral vectors (LV) produced were used more for HIV research than for gene therapy. The main aim was to prevent the production of a replication-competent lentivirus. The safety profile of LV has been improved through several generations. The first generation of vectors used a three plasmid system, splitting the trangene and the viral genes, to limit the possibility of generating a replication-competent vector. One of the plasmids contained the gene encoding the vesicular stomatitis virus glycoprotein (VSV-G) envelope protein, replacing the existing HIV envelope protein with that from another virus in a process termed “pseudotyping.” This increases the safety profile of the vector and can also alter the tropism of the vector. Pseudotyping can target the vector toward a specific cell type. The second generation of LV removed accessory genes from the viral genome which reduced the potential pathogenicity of the vector. Further refinement in the third-generation of lentiviral vectors resulted in removal of regulatory genes rev and tat. Rev allows unspliced mRNA to exit the nucleus intact. Tat functions in producing large amounts of mRNA transcripts which increases the transcription of the lentiviral genome. To remove tat , the viral long terminal repeat (LTR) was replaced with an LTR from an avian retrovirus. The rev gene was placed in a separate plasmid. This further reduced the possibility of a replication-competent lentivirus being produced. The fourth generation of LV are rev -independent vectors. However, replacement of the rev response element results in vectors titers lower than vectors produced with rev.
LV have been tested in preclinical studies in Parkinson’s disease where it has been used as a tricistronic vector. A single LV was used to deliver three genes that encode the rate-limiting enzymes involved in dopamine synthesis; tyrosine hydroxylase, aromatic amino acid dopa decarboxylase, and GTP cyclohydrolase I. This demonstrated efficacy in preclinical testing and has advanced to clinical trial. Phase 1 (NCT00627588) of this trial has been completed and it has met its primary safety endpoint. There was some indication of efficacy, but this will be more rigorously tested in a Phase 2 trial.
Herpes Simplex Virus
HSV is a large double-stranded DNA virus that is naturally neurotrophic. It is an enveloped virus with a subenvelope (tegument) and a viral capsid. HSV-1 can cause cold sores, keratitis and, in rare cases, encephalitis. HSV-2 is more associated with genital lesions and is considered more pathogenic. For development of viral vectors, HSV-1 has been used, as a less pathogenic virus was deemed more favorable.
HSV infects epithelial cells of the skin and mucosa via a lytic pathway. This allows de-enveloped progeny virus particles to infect the axons of sensory neurons in the affected region. The viral components are efficiently delivered to the nerve cell nucleus by retrograde axonal transport. They are transported to the neuron cell nucleus of either the dorsal root ganglion (DRG) or the trigeminal ganglion. The viral genome remains in the nerve cell nucleus as a latent circular episome for life. HSV natural viral latency has been found to be limited to neurons, which explains its neurotrophic nature. The latent virus can be reactivated in response to a recurring illness or immunosuppression. If this occurs, progeny viruses are produced and transported in an anteretrograde manner back to the nerve terminal to establish an infection at the original site of infection. However, this does not spread in individuals with a normally functioning immune system.
The development of HSV vectors has followed a similar pattern to other viral vectors, where the virus has been made replication deficient to reduce its pathogenicity. The first genes to be deleted were two of the immediate early (IE) genes. This allowed for the production of replication defective HSV vectors in cell lines that expressed the deleted genes. The vector retained the ability to transduce sensory nerves after intradermal delivery. A further advancement in HSV vector development was the identification of the latency gene (LAP2) which was positioned downstream of the latency promoter. The latency gene had independent promoter activity. LAP2 was found to express transgenes for extended periods of time. However, these vectors have limited lifetime in nonneuronal tissue and were cytotoxic to cells in culture. Production of HSV vectors that has the rest of the IE genes removed was achieved with creation of cell lines that expressed the deleted genes. This resulted in the viral genome being transcriptionally inactive and, therefore, the virus was nontoxic and persists in nondividing cells. Problems with transgene shutdown were overcome with use of an insulator sequence (CTRL) and an extended LAP2 (LATP2) to result in gene expression without any of the virus IE genes. This gave a vector capable of long-term gene expression that was not cytotoxic.
An alternative strategy was the creation of amplicon vectors. These are HSV vectors that contain an origin of replication and a packaging signal but have no lytic function. They are propagated using a replication-defective helper virus. Amplicon vectors can accommodate large transgenes (up to 100 kb) but are difficult to produce in high titers. Another option developed for HSV vectors was replication-competent vectors. These were developed with a view to treating cancer by delivering transgenes that activated anticancer drugs, induced innate killing mechanisms, and improved distribution of anticancer metabolites. Conditionally replicative vectors were developed that had genes contributing to neuropathogenesis removed. HSV replication-conditional vectors have been tested for their use in the treatment of cancer. This has been translated to the clinic and been applied in the treatment of glioblastoma and other forms of cancer.
HSV vectors have also been used in the treatment of chronic pain. They have been used to deliver proenkephalin, an antinociceptive gene, to the DRG in a preclinical pain model in rats. This resulted is alleviation of pain in these animals in response to a formalin injection. In a subsequent study, HSV-proenkephalin enhanced the effect of morphine in a spinal nerve ligation model and provided an analgesic effect in neuropathic pain. Further testing in a mouse model of bone cancer pain determined that HSV-enkephalin decreased pain-related behavior in these animals. This vector proceeded to phase 1 and 2 clinical trials (NCT00804076 and NCT01291901). The trials met its primary safety endpoint but did not proceed beyond Phase 2 testing.
Stem Cells
Stem cells are defined as cells that have the ability, under the correct conditions, to differentiate into a number of different cell types. Depending on the type of stem cell, they can differentiate into a wide variety of cell types or can be restricted to a few lineages. Stem cells have been extensively researched due to their therapeutic potential in regenerative medicine. The types of stem cells covered in this chapter include embryonic stem cells (ESCs), neural progenitor cells (NPCs), mesenchymal stem cells (MSCs), hematopoietic stem cells (HSCs), and induced pluripotent stem cells (iPS cells) ( Fig. 7.2 ).

Embryonic Stem Cells
ESCs are cells that differentiate into all of the somatic cells in an embryo. ESCs can be used to understand the mechanisms of development of cells and organ systems in the body. They also have great potential to be used therapeutically in regenerative medicine to replace damaged tissue and cells in patients.
Human ESCs are isolated from the inner cell mass of the human blastocyst. During normal development, they are present at day 4–7 postfertilization. After day 7, they begin to form the three embryonic tissue layers (ectoderm, mesoderm, and endoderm). Human ESCs were first grown successfully in a laboratory by Thompson et al . ESCs are termed “multipotent,” as they have the ability to become many different cell types. They express genes relating to transcription, chromatin remodeling, and DNA repair factors at higher levels when compared to differentiated cells. Research has shown that differentiation of ESC in vitro results in the down-regulation of pluripotency-associated genes.
There are a large number of studies that have differentiated ESCs into cells derived from the three germ layers. For example, endodermally derived cells include hepatocytes, lung epithelium, and insulin-producing β cells. Cells derived from the mesoderm resulted in the differentiation of chondrocytes, osteocytes, and skeletal myoblasts. Ectodermally derived cells include keratinocytes and NPCs. Functional motor neurons generated from ESCs in vitro were first reported by Li et al.
The first cells derived from ESCs, which advanced to clinical trial, were retinal pigment epithelium (RPE) cells to treat Stargardt’s macular dystrophy (NCT01345006) and dry age-related macular degeneration (NCT01344993). The RPE cells were administered via a submacular injection in a phase 1/2 open label trial. These trials were initiated in 2011 and first reported on in 2012. Initial follow-up on the trial participants reported no indication of rejection, tumorigenicity, hyper proliferation, or ectopic tissue formations at 4 months postsurgery. None of the patients’ vision deteriorated in this time. Longer-term follow-up reported safety in relation to RPE transplant and graft survival up to 22 months postsurgery. No adverse events were reported in relation to the transplanted cells. There were some improvements in vision but as this was an open label trial it is difficult to assess. For other clinical trials using ESCs see www.clinicaltrials.gov .
Neural Progenitor Cells
NPCs are tissue-specific stem cells that have been obtained from a postimplantation embryo. These cells have the capacity to differentiate into the three major cell types of the CNS (neurons, astrocytes, and oligodendrocytes). NPCs have some predetermined properties but their fate can be altered after exposure to certain external factors. Research has demonstrated that NPCs are spatially and temporally restricted in the profile of their gene expression. They are also limited in their ability to renew and differentiate. However, other studies have determined that NSCs lose some of their region-specific characteristics in vitro. External factors appear to play a significant role in determining what cell type the NSC will become. For example, basic fibroblast growth factor has been found to play a significant role in affecting the differentiating capacity of brain-derived NPCs and directing them toward a spinal motor neuron fate.
The potential for NPCs to be differentiated into neuronal cells types, such as motor neurons, and to be used to treat neurodegenerative diseases such as ALS and SMA is significant. The other therapeutic possibility is to use undifferentiated NPCs therapeutically. In theory, these stem cells could differentiate into cells that have been lost to disease progression. Alternatively, they could differentiate into support cells, such as astrocytes. This has been tested in preclinical models of ALS where it showed therapeutic efficacy and has been advanced to clinical trials. These studies are discussed in more detail in subsequent chapters.
Mesenchymal Stem Cells
MSCs are adult-derived stem cells. They were first isolated from bone marrow but have also been isolated from other adult tissues. MSCs are defined as multipotent and have the ability to differentiate into a number of different lineages (i.e., adipogenic, osteogenic, and chondrogenic).
Research has demonstrated that MSCs could be the foundation for an innate system of tissue repair. MSCs have been tested for their ability to affect tissue repair in animal models of a variety of diseases such as lung injury, kidney disease, graft versus host disease (GVHD), and myocardial infarction. It was also demonstrated that MSCs had a therapeutic effect on tissue repair despite a low or transient level of engraftment. For example, human MSCs injected into immunodeficient mice, with acute myocardial infarction, were undetectable 3 weeks postinjection. However, these mice continued to show improvement in cardiac function beyond this timepoint. This suggests that MSCs ability to secrete factors into the injured tissue may be a more important factor in tissue repair than their ability to differentiate into another cell type. MSCs, also, have well-documented immunomodulatory properties, with human MSCs having been found to express intermediate levels of major histocompatibility complex class I proteins. Other studies have found that MSCs have immunomodulatory effects on specific T-cell functions. This has been tested in experimental models of (GVHD). MSCs have also been shown to inhibit B cell differentiation.
One of the advantages of using MSCs as a cell therapy is the potential to isolate a patient’s own MSCs, expand them in vitro and deliver them as an autologous therapy. This would avoid the whole issue of rejection and the necessity for immunosuppression. The problem associated with this is the possibility of the MSCs, especially in genetic conditions, carrying the targeted disease.
A large number of clinical trials have been initiated using MSCs. These have delivered both autologous and allogenic MSCs as a therapy to treat a wide variety of diseases including diabetes, cardiomyopathy, and spinal cord injury. These trials have primarily been phase I/II trials that have focused on safety as their primary outcome. Delivery of MSCs has been demonstrated to be safe with different delivery methods. Some studies have reported promising efficacy data but this needs to be tested in a placebo-controlled, blinded study to verify these findings. For a complete list see www.clinicaltrials.gov . A number of trials have been conducted using MSCs as a treatment for ALS. These are detailed in a subsequent chapter.
Hematopoietic Stem Cells
HSCs are stem cells isolated mostly from bone marrow. They proliferate and differentiate giving rise to leukocytes and erythrocytes. Some HSCs circulate in the blood where they play a role in homeostasis and repopulate damaged bone marrow regions. Alternative sources of HSCs include peripheral blood and umbilical cord blood. Isolation from peripheral blood requires that HSCs are mobilized from the bone marrow into circulation. The can be achieved by administering hematopoietic cytokines, such as granulocyte colony stimulating factor (G-CSF), to the donor. Collecting HSCs from peripheral blood has the advantage of being less invasive that harvesting bone marrow, and this has become the preferred method of harvesting HSCs. Umbilical cord blood was first reported as a source of HSCs in 1982. The initial interest in cord blood was its potential to be used later in life as an autologous source of stem cells. However, with it being an easily accessed source of HSCs and the fact that it is tested and banked in advance, it is a valuable source for nonautologous transplants.
HSCs are primarily used in transplants to treat patients suffering from a range of hematologic diseases, particularly cancer. Patients receive a high dose of radiation or chemotherapy to ablate their endogenous HSCs. The HSCs are administered to repopulate the patient’s bone marrow. The first transplants were between twins which avoided any immune incompatibility problems. When they were expanded to allogeneic transplants, the issue of graft rejection or GVHD became apparent. Graft rejection was due to the host immune system attacking the transplanted cells. GVHD resulted from the host-reactive donor T lymphocytes attacking tissue in the recipient. It can cause major damage and be fatal if not treated. These problems led to a better understanding of the human leukocyte antigen complex and the need for immunosuppression, even in matched donors and recipients. T-lymphocyte depletion of the HSCs was found to reduce the risk of GVHD. However, this increased the risk of rejection and prolonged the time it took to for the transplanted cells to reconstitute. This resulted in these patients requiring additional support as they were vulnerable to infection for a prolonged period of time.
HSCs have also been used to treat diseases that do not have a hematopoietic origin. This includes diseases of the liver, heart, and brain. For example, it was noted that marrow cells that were infused intravenously into adult mice migrated to the brain. These cells were found to differentiate and express neuronal markers. These results were subsequently questioned due to conflicting data. Despite the conflict in the data, HSCs have been translated to clinical trials for stroke/ischemic brain injury. These trials have shown that HSC administration is safe but there is not enough data to demonstrate efficacy (see www.clinicaltrials.gov ).
Induced Pluripotent Stem Cells
iPS cells are adult-derived stem cells. They are developed by reprogramming somatic cells into a pluripotent state. This has been achieved in a number of different ways. One method reprogrammed somatic cells by transferring their nuclear content into oocytes. Another method fused an ESC with a somatic cell. These studies inferred that that certain factors in unfertilized eggs and ESCs were necessary for converting somatic cells into pluripotent stem cells. In 2006, Takahashi and Yamanaka conducted a study to identify these factors. They identified four factors that were necessary for induction of pluripotency. Two of the factors were found to be important in the maintenance of pluripotency in ESC and in early embryos, Oct3/4 and Sox2. The other two factors functioned in maintaining the ESC phenotype long term and also played a role in the proliferation of ESCs in vitro, c-Myc and Klf4. Somatic cells, fibroblasts, were transduced with these factors and then tested for their pluripotency. The cells were found to exhibit the morphology and growth pattern of ESCs. When they were injected subcutaneously into nude mice, teratomas were formed that contained all three germ layers. Researchers have continued to advance the protocol to develop iPS cells to use as alternatives to viral vectors. This includes episomal vectors, synthetic RNA replicons, human artificial chromosomes, transposon systems, and nanoparticle carriers. They have also looked for alternatives to the original four factors to induce pluripotency.
iPS cells can be developed from a patient’s fibroblast cells. One potential therapeutic use for iPS cells is to differentiate them into different cell types. These could then be transplanted back into the same patient, thereby circumventing the problem of rejection and the necessity for immunosuppression. iPS cells have been differentiated into a wide variety of cell types. This includes functional astrocytes, dopaminergic neurons, RPE cells, hepatocytes, cardiomyocytes, and red blood cells. A number of studies have been conducted to test cells in preclinical models of disease. One study transplanted NPCs, which had been derived from iPS cells, into the spinal cord of a rat model of ALS. These cells were found to survive for the duration of the study in both wild-type and in a rat model of ALS. The transplanted NPCs had differentiated toward a motor neuron fate and expressed motor neuron markers. How these cells would affect disease progression and life span requires further study.
The main problem with the autologous derived iPS cell therapeutic approach is as the somatic cells are taken from a patient the cells derived from them may carry the disease. This could limit the transplanted cells’ ability to be therapeutically effective. This is particularly true of genetic diseases. One way of exploiting iPS cells when dealing with a genetic disease is try and correct the defect in the stem cell. The corrected iPS cells can then either be administered or differentiated into the required cell type for treatment. This allows for use of autologous cells but eliminates the problem with genetic defects limiting therapeutic effectiveness. There are a number of genome-editing tools available that would allow for the correction of a mutation. These include zinc finger nuclease proteins, Clustered Regularly Interspaced Short Palindromic Repeats (CRISPR)—CRISPR associated (Cas9) (CRISPR/Cas9) system and Transcription Activator-Like Effector Nucleases. These genome-editing proteins use endogenous mechanisms for DNA repair within the cell and can be engineered to target a specific mutation.
iPS cells can be used to model diseases in vitro. This has been applied across a number of different diseases. In diseases, such as ALS, where a number of different genes can be linked to the disease, iPS cells have been created from a broad spectrum of patients. This allows for characterization of some of the underlying mechanisms of the different genetic causes of ALS and will assist is identifying where they overlap and diverge. This enhanced understanding will help in identifying new therapeutic targets. Another advantage of iPS cells is that they can be used to screen therapies in vitro before moving on to more expensive in vivo testing. This would be particularly useful for pharmacological agents.
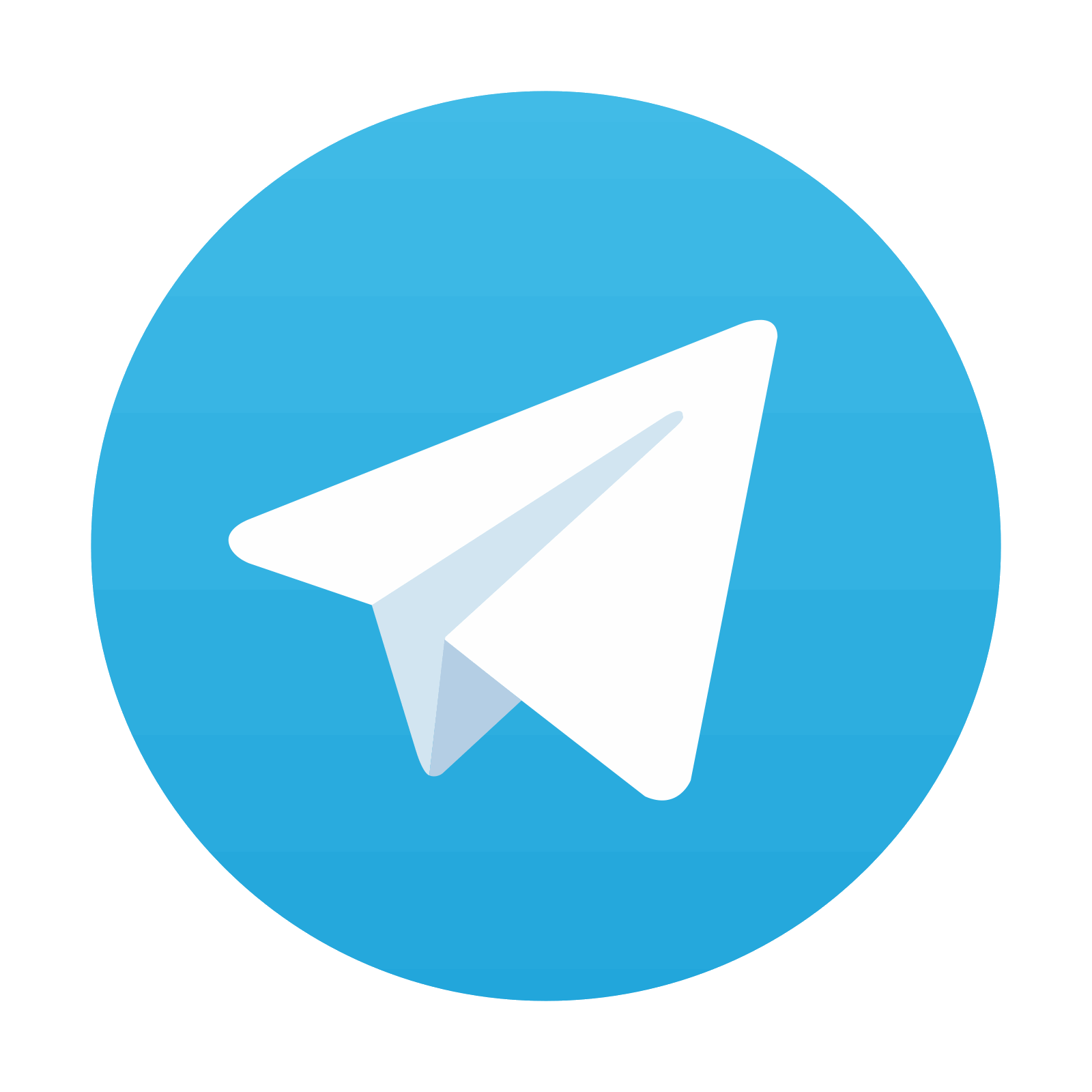
Stay updated, free articles. Join our Telegram channel
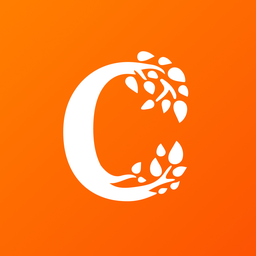
Full access? Get Clinical Tree
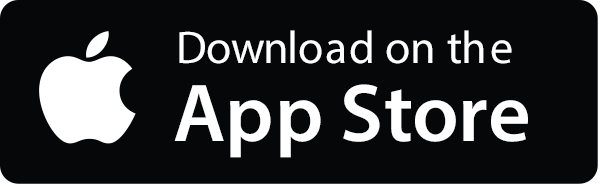
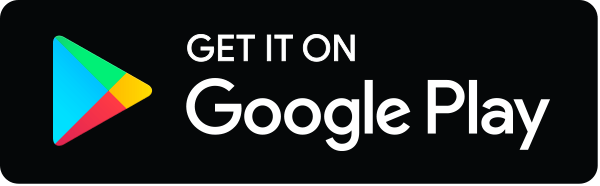