INTRODUCTION
Neurologic symptoms such as headache and low back pain are common reasons for patients to present to their primary care physician. Imaging plays a crucial role in the diagnosis of a wide range of neurologic disorders. However, radiology training in medical school or nonradiology residencies is sparse, and clinicians often have limited knowledge of different imaging modalities, imaging study appropriateness, and interpretation of emergent findings. Moreover, neuroimaging routinely employs advanced modalities such as magnetic resonance imaging (MRI) and is rapidly evolving in both technological developments and the understanding of imaging manifestations of pathophysiology. A significant number of these advanced imaging studies are requested by primary care physicians. Furthermore, overutilization of imaging resources has come under much scrutiny. The American College of Physicians emphasizes the appropriate use of diagnostic tests and has identified 37 situations in which a test does not reflect high-value care; these include three neuroimaging scenarios: imaging for low back pain, recurrent classic migraine, and simple syncope.
An understanding of imaging as it pertains to the diagnosis and management of neurologic disorders will aid the primary care physician in ordering the most appropriate examination, interpreting the radiologists’ reports, determining the next steps in management, and making appropriate referrals to specialists. The purpose of this chapter is to review the basic concepts of neuroimaging that are relevant to the primary care physician, specifically the appropriateness of imaging tests, typical imaging techniques and terminologies, factors to consider prior to contrast agent administration, and the imaging findings of common pathologies.
APPROPRIATENESS OF IMAGING
While the care of the individual patient is paramount, imaging resources are limited in most clinical environments. Certain imaging modalities are better suited to evaluate specific patient presentations or neurologic conditions, and responsible stewardship of these limited resources allows for better care of the entire community. Unnecessary or unwarranted imaging increases the burden of care on the patient, can be psychologically or emotionally stressful for the patient, and incurs additional financial costs. There are also small health risks to the patient with some imaging studies, such as the exposure to ionizing radiation from x-ray and computed tomography (CT) studies.
To help clinicians make informed decisions about imaging studies, the American College of Radiology (ACR) maintains an online list of Appropriateness Criteria ( https://acsearch.acr.org/list ). This resource summarizes the current literature regarding the role of imaging in the evaluation of common neurologic presentations and serves as a guide for choosing an appropriate imaging test. These recommendations assess the utility and safety of different imaging studies and rank them from most to least appropriate for each clinical scenario. Patient-friendly summaries of many Appropriateness Criteria recommendations are also available to download or print.
Multiple other organizations publish guidelines that include recommendations on diagnostic and interventional imaging studies specific to their field, such as the American Academy of Neurology ( https://www.aan.com/practice/guidelines ) and the American Heart Association ( https://professional.heart.org/en/guidelines-and-statements ). While the ACR Appropriateness Criteria attempts to synthesize the recommendations from other organizations, clinicians are encouraged to consider the recommendations that are best suited for their patient.
IMAGING TECHNIQUES AND TERMINOLOGY
Modern radiology offers multiple imaging modalities and techniques for the evaluation of neurologic conditions. In the primary care environment, CT is often the best modality for evaluating acute presentations. MRI is preferred for diagnosis or monitoring of chronic conditions, or in situations in which minimizing radiation exposure is important (e.g., pregnancy). Certain clinical presentations can also be well evaluated via ultrasound or nuclear medicine studies. Plain film radiographs (x-rays) have limited utility in neuroradiology outside of specific scenarios.
Computed Tomography
CT uses ionizing radiation (x-rays) to generate three-dimensional images of the body’s internal structures, generally correlated with their relative densities. Materials with significant density differences are easier to distinguish between (e.g., bone and air), while tissues with more subtle variations in density can appear less differentiated (e.g., gray matter and white matter). Compared to other types of imaging, the speed, ubiquity, and cost often make CT imaging the most accessible modality.
CT scans of the head and spine are often performed without contrast. Administration of iodinated contrast can be considered for patients who cannot undergo MRI if there is suspicion of intracranial metastases or spinal surgery complication such as infection. Spine CT with intrathecal contrast (CT myelography) is useful to assess the patency of the spinal canal and neural foramen in patients with radicular symptoms when MRI is contraindicated or nondiagnostic.
On head CT, common causes of increased density/attenuation (bright areas) within the brain are calcifications, hemorrhage, or contrast. Decreased density/attenuation (dark areas) is a nonspecific finding in the brain that can be due to infarct, mass, or edema. While the density difference between gray matter and white matter is subtle, careful adjustment of the imaging window can allow for the parenchymal characteristics to be discerned. Normal cerebrospinal fluid (CSF) is low density (dark) and surrounds the brain, which makes it useful for assessing the cortical sulci, brain symmetry, and ventricular size.
Magnetic Resonance Imaging
MRI uses strong magnetic fields and nonionizing radio waves to measure variations in the magnetic properties of tissue. The chemical composition and conditions within a tissue impart particular magnetic signatures that allow for imaging characterization. While a straightforward head CT scan can often be completed in a matter of seconds, the subtlety of the magnetic fields within the human body means that a brain MRI usually takes several minutes to complete. A patient who is unable to lie supine and motionless within the MRI machine for this duration of time may require additional accommodations (e.g., anxiolytic medications) or a differing imaging modality.
Because the strong magnetic fields of MRI interact with metallic objects within the body, certain precautions are necessary to avoid catastrophic outcomes. Electronic devices (e.g., pacemakers, pumps, stimulators) can malfunction, and small metallic objects (e.g., aneurysm clips, shrapnel, orbital foreign bodies) can become dislodged. Since the MRI magnetic field is variable and dynamic, a previous MRI of a different body part does not guarantee safety. The presence of a metallic object or device is not necessarily an absolute contraindication for MRI, however, and clinicians are recommended to refer to their institution’s guidelines or speak with a radiologist to clarify the risks and options.
By altering the magnetic fields and patterns of radio waves in an MRI, the characteristic signals from different tissues can be used to compose unique sequences or image types. Institutional protocols vary, but a brain MRI may include diffusion-weighted images (DWI), susceptibility-weighted images (SWI), gradient echo (GRE) images, T1- and T2-weighted images, and T2-weighted images with fluid suppression (T2-fluid attenuation inversion recovery [FLAIR]). MRI contrast agents have high T1 signal (bright), and additional postcontrast T1-weighted sequences are obtained in contrast-enhanced exams.
Areas of high signal (bright) on DWI with corresponding low signal (dark) on apparent diffusion coefficient (ADC) maps are referred to as areas of low or restricted diffusivity. These denote areas of infarction, pus, or high cellularity and are important for the evaluation of suspected stroke, infection, or malignancy. Given the high sensitivity of DWI for these critical pathologies, the DWI/ADC series will often be prioritized if the patient is unable to tolerate a complete MRI scan. In a similar vein, SWI/GRE images are particularly sensitive to metal such as iron or calcium and are useful for identifying areas of hemorrhage and calcification.
Spine MRI typically involves T1- and T2-weighted images, and a fat-suppressed T2-weighted sequence such as short tau inversion recovery (STIR). As in the brain, the postcontrast images will be T1-weighted sequences. Due to the geometric constraints of the strong magnetic field involved, MRI has an innate tradeoff between the size of the imaging field and the level of imaging detail. While this is not often an issue in the head, attempting to image the entire length of the spine all at once results in decreased image resolution and interpretability. The spine is therefore usually imaged in segments, and clinicians should provide guidance to the radiology team regarding the patient’s symptoms or area of interest within the spine to ensure optimum image quality.
Angiography
Both CT angiography (CTA) and MR angiography (MRA) can be used to assess intracranial vasculature. CTA requires administration of iodinated contrast and has the advantage of superior spatial resolution at the cost of radiation exposure. MRA is often performed based on the phenomenon of flow-related enhancement (called “time-of-flight MRA”) without the need for contrast administration. Importantly, a noncontrast MRA is only for assessment of vasculature and will not identify enhancing lesions.
Other
Although CT and MRI are the primary tools in neuroimaging and the most likely to be encountered by the clinician, ultrasound, nuclear medicine studies, and radiographs (x-rays) have certain specific and limited roles.
Beyond the neonatal period, ultrasound is not routinely employed to assess intracranial structures. Instead, ultrasound is primarily used in adult neuroimaging to evaluate carotid artery flow in the setting of transient cerebral ischemia or cerebral embolism.
Nuclear imaging studies also have specific functions in neurologic evaluation. The cortex avidly metabolizes glucose and so appears bright on 18F-fluorodeoxyglucose positron emission tomography (18FDG PET) studies. Some metastatic lesions, however, can display even greater avidity than normal brain tissue and therefore can be detected and followed on PET imaging. Gallium-68 radiotracers can be used to assess the patency of shunt catheters as well as for obstructive hydrocephalus. nuclear medicine DaTscans are routinely used to assess basal ganglia function in the diagnosis of parkinsonism. PET imaging can also readily display malignant lesions in the head, neck, and vertebral bodies of the spine.
Finally, plain film radiographs (x-rays) have a limited role in modern neuroimaging. Their primary roles are ruling out metallic objects in the orbits before MRI; osseus assessment of subacute, posttraumatic, or postsurgical lumbar back pain; and assessing the integrity of VP shunt catheters and shunt valve settings.
CONTRAST CONSIDERATIONS
Intravenous contrast is primarily used to highlight vascular structures and to better detect and characterize lesions. Because of the increased time, higher costs, and small risk of contrast extravasation or reaction, contrast-enhanced studies should be requested only for specific indications. CT contrast is best indicated for detecting intracranial metastases, vascular assessment (CTA), and postsurgical spinal infections. MRI contrast is useful for assessing tumors, infections, demyelinating disease, vascular pathologies, and posttreatment changes. If there are any questions about the appropriateness of a contrast-enhanced study, clinicians are advised to speak with a radiologist.
Renal Function
In nondiabetic patients without acute kidney injury (AKI) and with an estimated glomerular filtration rate (eGFR) greater than 30 mL/min/1.73 m 2 , there is little evidence that iodinated contrast media is an independent risk factor for AKI. There is, however, an increased risk of AKI in diabetic patients with eGFR less than 44 mL/min/1.73 m 2 . Neither threshold is an absolute contraindication to contrast administration, and intravenous fluids can be given prophylactically if needed. Patients with anuric end-stage renal disease on dialysis can safely receive iodinated contrast media, as there is no risk of further renal damage. There is no need to adjust the patient’s hemodialysis schedule following contrast administration. In patients on hemodialysis, patients with AKI, or patients with severe or end-stage renal disease (eGFR <30 mL/min/1.73 m 2 ) who are not on hemodialysis, certain classes of gadolinium-based MRI contrast agents (GBCA) are contraindicated due to the risk of nephrogenic systemic fibrosis.
Contrast Reactions
Reactions to iodinated contrast are rare (0.6%) and usually mild. The most common reactions are self-limited and resolve without progression, including nausea, vomiting, vasovagal reaction, pruritis, and cutaneous edema. Some of these reactions are purely physiologic (e.g., vasovagal reaction) while others are allergic-like (e.g., pruritis). Importantly, these reactions are not true allergies, in that they are not IgE-mediated. This means that there is no need for sensitization, and a reaction can occur to a patient’s first exposure.
The greatest risk factor for a contrast reaction is a previous reaction to the same class of contrast. In patients with a known allergic-like reaction to iodinated CT contrast media, steroid premedication should be strongly considered prior to administration. The ACR provides guidance on premedication regimens, though clinicians should refer to their institution’s guidelines. While patients with unrelated allergies (including allergies to shellfish and other iodine products) or asthma may be at increased risk of contrast reaction, the risk does not warrant steroid premedication.
Allergic reactions to GBCA are very rare (<0.2%), though in patients with a prior contrast reaction it may be prudent to premedicate with steroids and administer a different GBCA. There is no cross-reactivity between GBCA and iodinated contrast agents.
Pregnancy and Other Considerations
In instances when CT imaging is performed during pregnancy, the effect of iodinated contrast on the fetus is minimal, and it should be used when clinically indicated. The effect of GBCA on a fetus is unclear, and MRI contrast should be administered in pregnancy only when there is a potential for significant clinical benefit that outweighs the possible risk of fetal exposure. In these situations there should be a discussion between the clinician and patient regarding the risks, and informed consent is needed.
During breastfeeding, only a minuscule amount of contrast is excreted into breast milk and absorbed by the infant, so no precautions are needed for lactating patients. If desired, however, a lactating patient can abstain from breastfeeding for 24 hours following contrast administration and dispose of any milk produced during that time.
Gadolinium has been found to be deposited in the brain in patients who have received multiple doses of GBCA, though the clinical consequences of this are unknown and investigations are ongoing. There is also an uncertain relationship between iodinated contrast and exacerbation of myasthenia gravis symptoms. The iodine in CT contrast has a minimal effect on a normal thyroid, but iodinated contrast risks potentiating thyrotoxicosis in cases of acute thyroid storm.
IMAGING FINDINGS
Brain Pathologies
Stroke
In cases of suspected stroke, imaging is used to help decide whether a patient is likely to benefit from therapy. Treatment for acute embolic stroke often consists of thrombolysis with tissue plasminogen activator (tPA, alteplase) or thrombectomy. Intracranial hemorrhage, however, is an absolute contraindication to thrombolysis, as it may worsen the bleeding.
Emergent noncontrast CT of the head is performed prior to initiation of tissue plasminogen activator to exclude intracranial hemorrhage. Although hyperacute (<6 hours) infarcts are not reliably detected on CT, several imaging signs may be present to suggest the diagnosis ( Fig. 2.1 ). The insular ribbon sign is the loss of the gray-white differentiation along the insula and may be best appreciated lateral to the lentiform nucleus. Occasionally a dense vessel sign is identified at the site of vascular occlusion (often most apparent in the proximal middle cerebral artery [MCA]), in which a hyperattenuating (white) thrombus is seen within the vessel.

Acute hemorrhagic blood products appear hyperdense on CT imaging and are discussed in the section of this chapter titled “Trauma.” After excluding intracranial hemorrhage on a noncontrast CT, emergent noninvasive vascular imaging (CTA or MRA) is recommended prior to endovascular intervention to identify the site of occlusion. Administration of tPA, however, should not be delayed for vascular imaging. An abrupt cutoff of a vessel at the site of embolism with diminished or absent contrast perfusion of the distal vasculature may be most readily appreciated on maximum intensity projection imaging reconstructions ( Fig. 2.2 ).

Although MRI takes longer to perform than CT and is not suitable for all patients, DWI is the most sensitive imaging tool for detecting hyperacute infarcts (within minutes of ischemia). Areas of acute infarction display restricted diffusivity (DWI bright with matching ADC dark), without corresponding signal abnormalities on other sequences. As the ischemia progresses, other MRI findings can help to characterize chronicity. For example, T2/FLAIR hyperintensity is seen at >6 hours in the affected gray matter, and gyriform contrast enhancement is typically seen after 5 days.
Embolic occlusive infarcts most commonly affect the middle cerebral artery distribution. Small lacunar infarcts are due to occlusion of deep perforating arteries, most often within the basal ganglia, pons, internal and external capsule, and corona radiata ( Fig. 2.3 ). The presence of multiple bilateral infarcts in an anterior and posterior circulation distribution raises concern for a cardiac source of emboli ( Fig. 2.3D ), especially in patients with known arrythmias, cardiac wall motion abnormalities, or endocarditis. A right-to-left shunt (e.g., patent foramen ovale) can also allow emboli from venous thromboses to ascend into the cerebral circulation. Multiple unilateral anterior and posterior infarcts may be related to a fetal origin of the posterior cerebral artery, a common anatomic variant in which a large posterior communicating artery allows emboli to pass readily from the internal carotid artery into the posterior circulation ( Fig. 2.2B ).

Systemic hypotension in the presence of high-grade carotid stenosis/occlusion can result in “watershed” infarcts. These affect the border regions of the cerebral vascular territories, which are more susceptible to perfusional insufficiency. The most common areas are in the frontal cortex along the a nterior cerebral artery (ACA)/MCA border ( Fig. 2.3C ) and in the parieto-occipital region along the MCA/PCA border. Less commonly, a watershed infarction can occur at the triple border of the ACA, MCA, and PCA in the parieto-occipital region posterior to the lateral ventricles.
Perfusion imaging with CTA or MRA measures the affected ischemic core of nonsalvageable brain parenchyma and the surrounding penumbra of threatened tissue that may recover if it is reperfused ( Fig. 2.4 ). This is most useful for patients who are beyond the window for tPA (>6 hours) but who still may benefit from mechanical thrombectomy (<24 hours). Although the specific technique differs by vendor, perfusion imaging measures the volume and timing of cerebral blood flow to provide a color-coded map of the parenchyma that can assist in clinical decision-making.

CTA/MRA of the neck is often performed along with vascular imaging of the brain to assess for atherosclerotic disease of the carotid vessels and to create a map of the cervical vessels for potential endovascular intervention. Stenosis is quantified by using the North American Symptomatic Carotid Endarterectomy Trial or Asymptomatic Carotid Atherosclerosis Study criteria. Patients with symptomatic high-grade stenosis (>70%) are likely to benefit from carotid endarterectomy.
Ultrasound evaluation of the carotids can be conducted at the bedside to assess for stenosis using Doppler imaging to measure peak systolic velocity. Normal carotid flow is considered to be <125 cm/sec, with >230 cm/sec indicating >70% stenosis. Luminal narrowing and calcified plaques may also be directly visualized in real time on ultrasound.
In cases of severe or widespread ischemia, the imaging characteristics of diffuse anoxic/hypoxic injuries are similar to those of focal ischemia except manifested globally. On CT, there is a diffuse loss of the gray-white junction that is often most apparent along the bilateral insula. These changes are often delayed (>12 hours) compared to focal ischemia, and so a normal head CT shortly after an anoxic/hypoxic episode may be misleading. Additionally, diffuse cerebral vasogenic edema will result in sulcal and ventricular effacement. MRI imaging is again more sensitive in the acute period, though extensive diffusion restriction may be more difficult to identify without normal brain parenchyma for comparison.
Transient Ischemic Attack
Transient ischemic attack (TIA) presents as an acute functional neurologic deficit limited to a discrete vascular territory, secondary to a temporarily inadequate blood supply. Although classically a TIA was defined retrospectively based on the duration of the symptoms (<24 hours), up to 67% patients had evidence of acute infarction on imaging. Hence, the definition has been updated to “a transient episode of neurologic dysfunction caused by focal brain, spinal cord, or retinal ischemia, without acute infarction,” and brain imaging—preferably MRI—is recommended within 24 hours of symptom onset. Even if acute infarction is not present on initial imaging, there is an increased risk of progression to cerebral infarction within the acute to subacute period. Noninvasive imaging of the cervical and intracranial vessels (CTA, MRA, or carotid ultrasound/transcranial Doppler) should be routinely performed, as this has demonstrated ≥50% intracranial or extracranial stenosis in ~23% patients. Although TIAs have previously been associated with a high stroke risk of ~5% within 48 hours, aggressive management strategies have improved the prognosis. As many cases of TIA are likely missed due to their transitory nature, clinicians should have a low threshold for pursuing an MRI if there is clinical suspicion.
Trauma
Along with the physical and neurologic exam, imaging is one of the most critical components in evaluating patients following known or suspected head trauma. While some pathologies may manifest obviously on exam, imaging can identify conditions before they become critical. The clinical picture may also be complicated by multiple simultaneous traumatic injuries that can be identified and followed on imaging.
The CT and MRI appearance of blood products is related to their chronicity. On CT imaging, assessment of the evolution of blood products is based on density. In the hyperacute or actively bleeding stage, blood can appear isodense or hypodense (darker, gray) and may be difficult to identify compared to normal brain parenchyma and CSF. As the blood begins to clot over the next few minutes, however, it becomes denser (brighter, white) than the surrounding tissue. Two important corollaries are that (1) a thin rim of dense clot adjacent to bone may be challenging to identify, and (2) the blood of anemic patients will be less dense/bright. Continued bleeding leads to heterogeneity in the collection (swirl sign), and over time the blood products will separate into layers of clotted blood and serum. Eventually, the body will begin to resorb the blood, with the collection slowly decreasing in density over the course of several weeks to months.
On MRI, the appearance of hemorrhagic blood products is more complex and depends on the chemical state and metabolism of the iron within the hemoglobin. Hyperacute blood in the form of intracellular oxyhemoglobin (<6 hours) is T1 isointense (gray) and T2 hyperintense (bright). As the hemoglobin deoxygenates (6–72 hours), the blood signal becomes T1 and T2 hypointense (dark). The MR signal of the blood continues to evolve as the iron is metabolized into methemoglobin in the subacute period and eventually into ferritin and hemosiderin. As with CT imaging, a heterogeneous signal usually indicates blood products in various stages.
Extra-axial Fluid Collections
Blunt or penetrating injury to the head can result in hemorrhage into several compartments, including the epidural, subdural, and subarachnoid spaces ( Fig. 2.5 ).

An epidural hemorrhage/hematoma (EDH) is caused by bleeding into the potential space between the inner table of the skull and the dura mater ( Fig. 2.5A ). Because the dura adheres tightly to the cranium at the sutures, EDHs have a lens shape and do not cross the suture margins. The most common etiology (90%) is laceration of the middle meningeal artery secondary to temporal bone fracture (which itself will be best evaluated on CT). The classic presentation of a lucid interval followed by loss of consciousness is seen in up to 50% of patients with EDH. This delay occurs as blood gradually accumulates within the epidural space before causing rapid midline shift and brain herniation. Thus a suspected EDH warrants prompt recognition and close follow-up, as it may require surgical evacuation. Venous EDHs are much less common, and a small venous EDH in the anterior aspect of the middle cranial fossa is likely to be indolent.
A subdural hemorrhage/hematoma (SDH) is caused by bleeding into the potential space between the dura mater and the arachnoid mater ( Fig. 2.5B ). SDHs are crescentic, can cross suture lines, and may extend along an entire cerebral hemisphere. Although they can involve the falx, they will not cross the midline. SDHs are usually caused by tearing of the bridging veins. This is more common in elderly patients in whom parenchymal volume loss has stretched the veins, and it may be exacerbated by systemic anticoagulation. Unfortunately, that same parenchymal volume loss may also lead to delayed diagnosis, as the SDH has greater room to expand before becoming symptomatic from mass effect. Isodense or bilateral symmetric SDHs can be challenging to identify, since they can appear similar to brain parenchyma and may not cause midline shift. In elderly patients with normally prominent sulci, the effacement (compression or absence) of the sulci should warrant extra attention.
A subdural hygroma is a collection of (nonblood) fluid within the potential space between the dura mater and the arachnoid mater. Subdural hygromas may be due to a tear in the arachnoid mater that allows CSF to accumulate in the subdural space, or they can represent the fluid remnants of a degenerated chronic SDH. They are often difficult to differentiate from normal CSF on CT imaging with their similar densities, though are more apparent on MRI because of the higher protein content in the hygroma (FLAIR bright) ( Fig. 2.5C ). While most subdural hygromas are asymptomatic, intervention may be warranted if there is evidence of mass effect.
A subarachnoid hemorrhage (SAH) is caused by bleeding into the space between the arachnoid mater and the pia mater ( Fig. 2.5D ). Trauma and ruptured aneurysm are the most common causes of SAH, with nontraumatic SAHs classically presenting as a “thunderclap” headache. Blood in a SAH will infiltrate along the sulci, with larger hemorrhages extending into the cisterns and fissures. The pattern of the SAH can indicate the site of aneurysm, such as hemorrhage in the anterior interhemispheric fissure (anterior communicating artery) or in the suprasellar cistern (posterior communicating artery), though blood may redistribute with time and patient positioning. There are multiple complications of SAH that are well assessed on imaging, including hydrocephalus and vasospasm. Hydrocephalus occurs when clotted blood obstructs arachnoid granulations and impairs CSF resorption. It can result in brain herniation and may warrant ventricular shunt placement. Vasospasm occurs around 7 to 10 days following SAH and can result in cerebral ischemia. Although monitoring of vasospasm is best done via interventional angiography, CTA of the head is often a more practical method.
Intraventricular hemorrhage (IVH) can occur from a number of injuries and is most often secondary to direct extension of a SAH or intraparenchymal hemorrhage. Primary IVH may occur from the tearing of subependymal veins, vascular malformations, or intraventricular tumors. Although IVHs are often secondary to other trauma ( Fig. 2.5E ), they are particularly important to note because of the risk of a clot causing obstructive hydrocephalus.
Traumatic Brain Injury
Primary traumatic brain injuries include cortical contusion, intraparenchymal hematoma, and diffuse axonal injury. The coup-contrecoup injury pattern most commonly manifests with cerebral contusions and traumatic subarachnoid hemorrhages. In the coup-contrecoup pattern, soft tissue and fracture injuries occur at the site of initial impact and brain contusion/hemorrhage occurs at the opposite side of the head (e.g., left frontal and right parietal) ( Fig. 2.6 ). Any visible brain injury at the site of the initial impact is often less severe than that on the opposite side. Therefore identification of the site of trauma is important, and an injury anywhere in the brain should prompt a close examination of the opposing site where the brain impacts the inner skull.

Cortical contusions are the most common primary traumatic brain injury. They occur from blunt head trauma resulting in the collision of the brain against the inner table of the skull. Because of their etiology and the irregular shape of the skull base, they are most common in the frontal lobes along the anterior cranial fossa and the anterior temporal poles along the middle fossa. In the hyperacute setting, these injuries can be difficult to see on CT and may manifest only as small hypodense or hyperdense subcortical irregularities. As the contusions slowly bleed over the course of hours to days, however, the intraparenchymal hematomas (IPH) may become more apparent on CT. Focal areas of high density (white) surrounded by low density represent the clotted blood products with adjacent parenchymal edema ( Fig. 2.7A ). Although MRI is not often used for assessing acute injuries, it can be more sensitive within the first 24 hours and will demonstrate hypointense (dark) foci on SWI/GRE sequences at the sites of microhemorrhages. Diffuse axonal injury (DAI) results from shearing due to differential motion of the gray and white matter during acceleration and deceleration and typically results in loss of consciousness at the time of impact. Initial head CT is often negative, so DAI should be suspected in a patient with discrepant clinical and imaging findings with a reported mechanism of severe trauma. MRI dark foci on SWI/GRE often identify sites of DAI at the gray-white junction, splenium and posterior body of corpus callosum, brain stem, basal ganglia, internal capsule, and superior cerebellar peduncle ( Fig. 2.7B ).
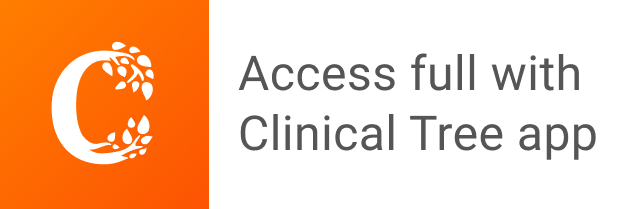