Background: radiation biology and physics overview
Radiation therapy treats cancer by causing DNA damage, most importantly double-strand breaks (DSBs). Although normal cells can efficiently detect and repair DSBs through homologous recombination or non-homologous end joining, malignant cells lack these mechanisms and DSBs are often lethal. As a result, malignant cells are more susceptible to radiation-induced DNA damage than normal cells. Importantly, normal cells can safely tolerate only a finite dose of radiation without risk of permanent damage. Radiation therapy is designed to maximize dose to the tumor while minimizing dose to normal surrounding structures.
Radiation dose represents the energy absorbed per unit mass, Gray (Gy). Alternatively, radiobiological equivalent dose, Gy(RBE), may be used to quantify biological damage increases resulting from different types of radiation. The total dose of radiation is typically divided into smaller daily doses, a technique termed fractionation. There are several biologic rationales to support fractionation of radiation therapy. Most importantly, fractionation provides opportunity for normal tissues to repair radiation-induced DNA damage, thereby reducing toxicity. Fractionation also allows time for malignant cells in relatively radioresistant phases of the cell cycle (S phase) to cycle to more radiosensitive phases of the cell cycle (G2 and mitosis). During the course of fractionated radiation therapy, hypoxic niches of the tumor are reoxygenated, thereby making those cells more sensitive to radiation.
Despite advances in radiation techniques that minimize dose to normal tissues, a number of early and late toxicities are common. Early radiation toxicity (during or within weeks of radiation treatment) occur in rapidly dividing tissues. Radiation accelerates cell loss while impairing cell proliferation, disrupting a precise equilibrium. Early radiation toxicity is generally reversible after completion of radiation treatment and return of equilibrium between cell replication and loss. Common examples include hair loss and mucositis but are dependent on the site irradiated. Late radiation toxicity occurs due to permanent loss of organ parenchymal cells and radiation-induced signaling (such as TGFβ) that promotes fibrosis. Examples include infertility, coronary artery disease, and bowel malabsorption. Late toxicities are generally irreversible. Rarely, radiation therapy may also cause a second primary tumor that can be benign or malignant, depending upon the irradiated tissue. Radiation exposure induces a mutator phenotype in which cells more readily acquire additional mutations that may be oncogenic. The risk of secondary malignancy is higher in patients treated at younger ages (i.e., younger age confers increased risk due to longer follow-up duration), higher cumulative dose, site of irradiation, receipt of chemotherapy, and in those with tumor predisposition syndromes (e.g., neurofibromatosis; see Chapter 16 for further discussion of neurofibromatosis).
Types of radiation therapy
Radiation therapy is most commonly delivered from a source outside the patient, an approach termed external beam radiation therapy (EBRT). Radiation oncologists use several techniques to plan and deliver EBRT. Three-dimensional conformal radiation therapy (3D-CRT) refers to radiation planning that relies on high-definition CT images for planning, often with additional assistance by MRI. There are several types of advanced 3D-CRT techniques that enable more precise delivery of radiation. The implied form of 3D-CRT is the original that employs software that enables the planner to place any number of beams of radiation from different directions, custom-shaped from the sides, and with some ability to attenuate the beam with materials placed in the path of the beam. More common forms of radiation delivery that also require the same 3D-based planning CT scan, but which use different principles and more advanced software to plan, are intensity modulated radiation therapy (IMRT) and volumetric modulated arc therapy (VMAT). IMRT and VMAT are complex delivery techniques that use software optimization techniques to determine the ideal treatment parameters using multiple beam segments with nonuniform dose intensities that add together to create treatment plans that meet predefined constraints and objectives. The most desirable treatment plans deliver the prescribed radiation doses to the target (the tumor, tumor bed, or areas at risk of disease recurrence as defined by the radiation oncologist) while minimizing dose to surrounding normal tissues (prioritized for dose-sparing as defined by the physician). Multiple static beams (often 5–9 beams and sometimes more) are used with IMRT, whereas one or more arcs are used with VMAT, which delivers the radiation therapy using equipment that rotates around the patient.
Stereotactic radiosurgery (SRS) is a type of EBRT that delivers the entire dose of radiation in one single or several small fractions of RT. SRS may be delivered with any high conformity technique but most commonly utilizes multiple radiation arcs or beams to converge on a precisely defined target. This optimizes treatment to deliver high doses to a confined area with little radiation exposure to adjacent tissue (i.e., no margin). Radiation dose to critical intracranial structures must be carefully considered during the planning process. Radiation dose efficacy on tumor and tolerance of normal tissues is extremely dependent upon the number of fractions (e.g., how interspaced) used to deliver the RT dose. Cranial irradiation is complex because of the multiple radiation sensitive structures including the optic chiasm, optic nerves, cochlea, hippocampus, brainstem, pituitary, retina, lacrimal gland, and lens. All EBRT techniques require careful patient positioning and target localization for treatment.
Radiation therapy most commonly utilizes photons but increasingly may utilize protons in certain clinical scenarios. Photons, or high energy x-rays, are generated by linear accelerators. Photons can also be harnessed in the form of gamma-rays emitted by multiple low-energy Cobalt-60 radioactive sources for SRS. In contrast, protons are generated from proton accelerators that are larger and more complex than linear accelerators. The therapeutic advantage of protons is not in the type of RT delivered, which is essentially the same for protons and photons, but in reducing damage to normal tissue around the tumor. The physical properties of protons allow for reduced dose of radiation to normal tissues adjacent to the tumor while maintaining therapeutic dose delivered to the tumor. The clinical benefit of protons relative to photons is thus far widely accepted for a number of diseases including uveal melanoma, skull base malignancies, sinonasal malignancies, and childhood malignancies such as medulloblastoma, craniopharyngioma, and rhabdomyosarcoma. The benefit of proton therapy for many other disease sites (such as breast cancer and prostate cancer) remains an area of ongoing investigation. The main disadvantage of proton therapy is the expense of establishing and operating proton facilities, which has historically limited its availability.
Clinical cases
Case. A 65-year-old male presented with left facial droop, difficulty ambulating, and emotional lability. An MRI showed a heterogeneously enhancing, well-circumscribed right temporal mass measuring 6.5 × 5.1 × 4.3 cm 3 with mass effect causing 1.1 cm leftward midline shift and uncal herniation ( Fig. 3.1A ). The patient underwent a craniotomy with near gross total resection of the tumor. Pathology showed WHO grade IV glioblastoma. Molecular analysis showed MGMT promoter hypermethylation, IDH1 wildtype, ATRX retained, and TP53 mutation. Postoperative imaging showed a residual nodular focus of enhancement and persistent fluid attenuated inversion recovery (FLAIR) hyperintensity surrounding the resection cavity. Following resection, the patient’s neurologic deficits completely resolved, and he had an excellent performance status. The patient was treated with adjuvant photon EBRT to the resection cavity with a total dose of 60 Gy in 30 fractions (2 Gy per fraction) over 6 weeks ( Fig. 3.1B ). The patient was also treated with concurrent and adjuvant temozolomide chemotherapy. The patient tolerated treatment well with only fatigue, mild appetite loss, and expected patchy partial alopecia.

Teaching Points. The standard of care for high-grade glioma is maximal safe resection followed by combined modality therapy consisting of adjuvant radiation therapy and temozolomide. Because of the infiltrative nature of high-grade glioma, microscopic disease extends beyond the radiographically defined tumor into the adjacent radiographically normal appearing brain parenchyma. As a result, radiation is delivered to the resection cavity plus a margin of adjacent brain parenchyma (most commonly between 1.0 and 2.5 cm depending on the physician) to provide coverage of infiltrative tumor cells. The typical radiation course for high-grade glioma is 60 Gy delivered in 30 fractions (2 Gy per fraction) over 6 weeks (Monday–Friday, no weekend or holiday treatments). Radiation can be planned and delivered with several EBRT techniques, including 3D-CRT, IMRT, and VMAT. Among patients with high-grade glioma, adjuvant radiation therapy improves disease control and survival but is not curative.
A variety of treatment approaches should be considered for patients who are elderly or debilitated. Among the elderly (generally defined as over the age of 60 or 65) and those with poor performance status, a shorter course of radiation therapy with concurrent and adjuvant temozolomide is well tolerated. These hypofractionated regimens include 40 Gy delivered in 15 fractions over 3 weeks or even 25 Gy delivered in 5 fractions over 1 week. Among patients who cannot tolerate combined modality therapy (i.e., radiation and chemotherapy), treatment with either radiation or temozolomide monotherapy should be considered depending on MGMT methylation status. Patients with MGMT unmethylated disease benefit greater from hypofractionated radiation therapy monotherapy over temozolomide monotherapy. In patients with MGMT methylated disease, temozolomide monotherapy can be appropriate in selected cases.
Follow-up MRI should be performed 1 month after completion of radiation therapy and then every 2 to 4 months thereafter. Initial imaging 1 month after radiation therapy establishes a new baseline prior to continuation of additional adjuvant chemotherapy. It is important to consider the possibility of pseudoprogression (treatment-related imaging changes that resemble disease progression) in patients with apparent disease progression within the first several months after radiation therapy. In patients with pseudoprogression, imaging changes and new clinical symptoms will typically resolve without additional cancer-directed therapy. Patients with MGMT methylated glioblastoma treated with radiation therapy and concurrent temozolomide are at increased risk of pseudoprogression. In these cases, serial imaging is recommended as advanced MRI-based imaging techniques, positron-emission tomography (PET) imaging, or other noninvasive tools cannot reliably differentiate pseudoprogression from true tumor progression. In selected cases, surgery may be needed (see Chapter 2 for approaches to surgery in brain tumor patients).
There are multiple acute and late toxicities of radiation therapy for high-grade glioma. The most common acute toxicities are alopecia, radiation dermatitis, fatigue, and loss of appetite. These toxicities are typically mild, managed with supportive care, and resolve after completion of radiation therapy. Late toxicities occur months to years after radiation therapy, may be irreversible, and most commonly include neurocognitive impairment, leukoencephalopathy, and endocrinopathies (e.g., thyroid disease).
Clinical Pearls
- 1.
The standard of care for high-grade glioma is maximal safe resection followed by combined modality therapy consisting of adjuvant radiation therapy and temozolomide. The typical radiation regimen is 60 Gy in 30 fractions (2 Gy per fraction) delivered over 6 weeks.
- 2.
Among the elderly or those with poor performance status, hypofractionated radiation therapy (for example, 40.05 Gy in 15 fractions delivered over 3 weeks) with temozolomide, radiation therapy alone, or temozolomide alone may be appropriate in certain patients. This decision is often guided by MGMT methylation status.
- 3.
EBRT for high-grade glioma may be planned and delivered with 3D-CRT, IMRT, or VMAT.
- 4.
Imaging follow-up should occur 1 month after completion of radiation therapy to establish a new baseline prior to continuation of additional adjuvant chemotherapy. The possibility of pseudoprogression instead of early disease progression should be considered, particularly in patients treated with concurrent temozolomide.
Case. A 39-year-old female never-smoker presented with hemoptysis and a right hilar mass on imaging. Bronchoscopy showed lung adenocarcinoma that was positive for the ROS1 translocation. Several days later, the patient developed left arm clumsiness and headache. MRI imaging revealed five small brain metastases, diffuse osseous metastases in the spine and ilium, and multiple hepatic metastases. The patient was treated with crizotinib and pemetrexed with good response including in the brain. Approximately 1 year later, routine staging brain MRI showed a punctate focus of enhancement in the right cerebellum which subsequently enlarged to 5 mm in size on follow-up imaging. This was the only site of disease progression. At the time of evaluation for radiation therapy, the patient was asymptomatic from the brain metastasis and neurologically intact on examination. She had excellent performance status limited only by fatigue related to systemic therapy. The lesion was treated with single fraction SRS of 18 Gy ( Fig. 3.2 ). Follow-up MRI 2 months after SRS showed complete radiographic resolution of the right cerebellar metastasis, but a new punctate enhancing lesion appeared in the right temporal lobe. The right temporal lesion enlarged to 4 mm on imaging 4 months later. The right temporal lesion was then treated with SRS to 18 Gy ( Fig. 3.2 ). The patient experienced no side effects from either SRS treatment. Neither brain metastases recurred on follow-up imaging available 2 years post-treatment.
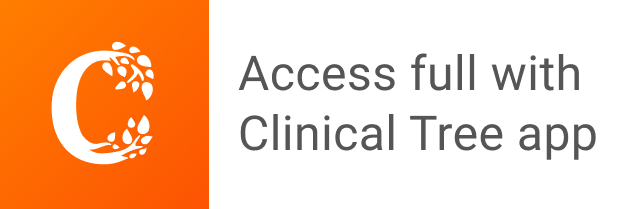