1939 Invasive Video-EEG The placement of electrodes intracranially to record electroencephalography (EEG) directly from the surface of the brain was pioneered by Foerster in the 1930s. Invasive EEG monitoring remains an essential tool for defining the epileptogenic zone in around 30% of patients considered for epilepsy surgery. Today, both these techniques – subdural and intracerebral stereotaxic EEG (SEEG) – remain in wide use in the presurgical evaluation of potential candidates with drug-resistant focal epilepsies. Regular communication between the medical and EEG technical team and hospital clinical engineering departments can do much to assure the quality of data acquired from intracranial EEG while keeping patients safe in an often electrically complex environment. Analysis of the interictal EEG from invasive recordings is an essential prerequisite for proper interpretation of the ensuing ictal data. Electrical stimulation mapping represents an integral part of any invasive evaluation, whether subdural grid electrode or SEEG. data acquisition, data interpretation, electrical stimulation mapping, epilepsy surgery, epileptogenic zone, invasive video-electroencephalography, presurgical evaluation, technical considerations Electric Stimulation, Electroencephalography, Epilepsy, General Surgery The placement of electrodes intracranially to record EEG directly from the surface of the brain was pioneered by Foerster in the 1930s. Two decades later, the technique of brain-penetrating electrodes that chronically recorded from superficial and deep cortex was developed by Talairach and Bancaud. Today, both these techniques—subdural and intracerebral stereotaxic EEG (SEEG)—remain in wide use in the presurgical evaluation of potential candidates with drug-resistant focal epilepsies. In general, an intracranial evaluation is undertaken when there is some—but insufficient or discordant—localizing evidence on the noninvasive evaluation to accurately identify a single epileptogenic zone (EZ; or network) amenable to surgical resection. For instance, the noninvasive data may point to a right temporal lobe epilepsy, but leave open the issue of the posterior extent of temporal lobe involvement, or the relative roles of the mesial and lateral temporal lobe. The purpose of the evaluation is then to explore the relevant brain areas at closer physical proximity, with the aim of more precisely delineating the extent and boundaries of the EZ. A related, though different, purpose of intracranial evaluation can be to delineate eloquent cortex from areas of seizure onset and early propagation. In this way, intracranial evaluation offers granular data to design an appropriate resective surgical procedure that maximizes benefit—by removal of the EZ—and minimizes the risk of neurological deficit—by respecting boundaries that define eloquent cortex. FIGURE 9.1. Preoperative implant schema and postoperative visualization. (A) Left fronto-temporal subdural grid electrode (SDE) implant plan with grids and strips covering the left temporal, posterior temporal, lateral frontal, orbitofrontal, and basal temporal surfaces. (B) Actual electrode positions identified from postoperative CT shadows and superimposed in a surface rendering of the left hemisphere from a volumetric preoperative MRI. Only the lateral brain surface is shown for clarity. (C) Postoperative skull x-ray confirming implant positioning as intended. (D) Implant plan for stereotaxic EEG (SEEG) exploration of a patient suspected to have a left lateral neocortical temporal lobe epilepsy. Acronyms refer to electrode targets (PIN = posterior insula, AH = anterior hippocampus, etc.) with the leading “L” referring to the left hemisphere. (E–F) Actual electrode positions obtained with the same procedure as (B) above. The lateral view shows the entry of multiple orthogonally placed trajectories; the coronal view shows the positioning of the entire left amygdalar electrode; also seen end-on is a single contact of the posterior insular electrode within the insula (arrow). TECHNICAL CONSIDERATIONS The deliberate formulation of an implantation plan is mandatory prior to intracranial exploration. Planning normally occurs by consensus during review of the relevant noninvasive data in a multidisciplinary conference attended by the neurology and neurosurgery teams. For a subdural grid electrode (SDE) implant, the laterality, lobar, and sub-lobar regions of coverage are determined; these subsequently guide the craniotomy and brain exposure and specific grid geometries utilized (Figure 9.1 A–C). For SEEG, planning is a more nuanced process, with specific enumeration of particular anatomical structures to be sampled and the electrode trajectories utilized for doing so in a safe manner (Figure 9.1 D–F). FIGURE 9.2. Commercial intracranial electrodes (PMT Corporation, Chanhassen, Minnesota). (A) 20-contact subdural grid electrode, platinum–iridium alloy, contact diameter 3 mm, inter-contact center-to-center spacing 10 mm, thickness 0.76 mm. (B) Depth (SEEG) platinum–iridium alloy electrode with removable stylet, 10-contacts of length 2 mm each, outer diameter 0.8 mm, 3.5 mm center-to-center spacing. Electrodes themselves are made of stainless steel or a platinum–iridium alloy. The latter has the advantage of being MRI-compatible, in the event of postimplant MR imaging being required. For SDE, electrodes are discs of 2 to 5 mm diameter, embedded in flexible Silastic® and spaced apart by 5 to 10 mm. Vendors provide a range of geometries, such as longitudinal strips or rectangular sheets of varying sizes. The standard geometries can also be cut and shaped appropriately to match the contour of the exposed cortical surface at craniotomy. SEEG electrodes are cylindrical contacts: length 2 to 2.5 mm, diameter 0.8 to 1.2 mm, embedded along flexible Silastic® catheters, 2 to 5 mm apart (Figure 9.2). The surface area of the contacts for commonly used SEEG electrodes range from 0.04 to 0.08 cm2 and 0.03 to 0.2 cm2 for subdural electrodes. Following electrode implantation, accurate visualization in situ is necessary. This is usually accomplished by thresholding a postimplant CT to reveal just the electrodes, and then fusing the thresholded CT volume with the preoperative volumetric MRI. Image fusion is available on several clinical picture archiving and communication (PACS) systems, though many centers will perform this step offline with commercial or in-house software (Figure 9.1 B, E–F). FIGURE 9.3. Sequence of connections for intracranial recording. (A) Subdural grid electrode placed over the right hemispheric convexity (arrow) whose output is joined to the connector clip (double arrow) of a cable that plugs into an EEG jackbox (triple arrow). The order of the jackbox connections follows a predetermined plan that reflects the number ordering of the contacts on the grid; (B) The output cable from the jackbox (arrow) connects to the input port of the EEG amplifier (circle); (C and D) The output from the amplifier (circle) connects through a USB port to the acquisition computer (arrow); (E) The acquisition montage is built within the EEG software interface. Connecting the externalized wires from SDE and SEEG implants to EEG review systems is a multi-step process (Figure 9.3) that is prone to human error. Systematized institutional protocols that specify the personnel qualified to do so (usually senior registered EEG technologists), together with checks and balances (such as independent review of the connections by other technologists and/or the attending medical team) are advisable. In essence, each individual SDE grid or SEEG electrode has exit wires that are uniquely numerically (and color) coded. The numeric/color codes are methodically paired with their particular implant locations during surgery. Following the implant, the numeric and color codes on the exit wire are the only indication of where its associated intracranial contacts are located. For SEEG, since each electrode exit wire comes out through its own hole in the skull, it is still possible to pair a particular exit wire to its intracranial electrode location after the fact. For an SDE implant, this is impossible due to all the exit wires coming out together through a single scalp location. The initial step of the connection process uses purpose connector clips to attach the exit wires from the patient to the EEG acquisition jackbox. The connections to the jackbox proceed in strict numerical sequence, so that the correspondence between the jackbox contact number and a particular electrode contact is predictable and intuitive. The jackbox exit cable connects to the input port of the EEG amplifier, which in turn connects to the acquisition computer. Montages are built within the software hosted by the acquisition computer, where the numbered jackbox contacts are retranslated into acronyms that indicate the brain region corresponding to the individual electrode contacts. FIGURE 9.4. Circuitry for use of a software-controlled electrical stimulator (arrow, Natus Medical; Pleasanton, CA). The stimulator is powered by a direct current (DC) supply unit (double arrow) and delivers output through its connection to the jackbox (triple arrow). The EEG software “opens” the contacts to be stimulated (though a separate cable connection to the jackbox—not shown) at the moment of stimulation, with the parameters of stimulation adjusted by the controls on the stimulator. Stimulation is delivered and aborted by the green start and red stop pushbuttons. Prior to beginning acquisition, a reference electrode should be specified and placed. In principle, any intracranial contact may be specified as the reference, since the data can be digitally rereferenced once acquired. It is prudent however to make a considered initial choice of reference that is believed to be far from area of pathology and as “inactive” as possible. For SEEG, the ideal reference would be a white matter contact distant from pathological brain areas. Due to the high amplitude of intracranial EEG in comparison to scalp EEG, an extracranial source (i.e., scalp EEG contact) can also be used. In addition to the reference electrode, one of the recording contacts should also be specified as the ground electrode. In patients needing cortical stimulation mapping, electrical stimulators are an addition to the configuration of the recording circuitry (Figure 9.4). Easiest to use are software-controlled stimulators. These are stand-alone units powered by direct current (DC) connecting to the jackbox. A menu in the EEG review workstation specifies the channels to be stimulated. Dials on the stimulator specify the parameters of electrical stimulation train, and a pushbutton delivers the stimulus itself. Sources of electrical noise in the recording environment are ubiquitous. Some centers will manage their intracranial patients within an intensive care unit, where electrical noise can be more problematic than on a normal hospital ward. Issues of electrical safety are also particularly germane in patients undergoing intracranial EEG recording, whose brains are in direct contact with the environment via multiple low-resistance pathways. Regular communication between the medical and EEG technical team and hospital clinical engineering departments can do much to assure the quality of data acquired from intracranial EEG while keeping patients safe in an often electrically complex environment. 198DATA ACQUISITION AND REVIEW Data Acquisition Digital invasive video-EEG is recorded with a sampling rate of at least 256 Hz, which can sufficiently collect low-voltage fast activity (LVFA; beta and gamma) often characterizing the seizure onset. A minimum of 128 channels is recommended for intracranial recordings but 256 is preferable. A video camera which can be controlled through the network and synchronized to the EEG allows capturing the patient’s clinical symptoms and their evolution. The camera should provide sufficient resolution for close-up recording and infrared capabilities for nighttime. Simultaneous recording of SEEG and scalp EEG can be safely performed. Higher sampling rates of 1 to 2 kHz are required to resolve high-frequency oscillations (HFOs; 80–250 Hz: ripples) or very high-frequency activity (250–500 Hz: fast ripples). For visual analysis of HFOs, a highpass finite impulse response filter (FIR) is applied at 80 Hz for ripples and 250 Hz for fast ripples. Other filters, like the infinite impulse response filter (IIR), should be avoided as they can cause false positive ripples (by “ringing”). Visual analysis requires an extended time scale of 0.5 to 2 seconds per page and a gain setting between 1 and 5 μV/mm. Ultra-slow activity, or “DC-shift,” requires specialized amplifier and nonpolarizable electrodes. However, slow potential shifts (SPS) up to 0.01 Hz can be recorded on standard amplifiers by reducing the low-frequency filter to its least value. Data Review Local storage capacity should be sufficient to accommodate the high volume of recorded data. Robust high-speed Internet connectivity is essential for data transfer and review. Large digital screen displays with sufficient spatial resolution are needed to accommodate the large number of channels and allow visual recognition of high-frequency activity. The recording is visualized in referential and in bipolar montage using adjacent contacts. For stereo EEG, it is useful to examine activity at individual electrodes to separate gray and white matter contacts. A selection of the most informative contacts by region can be used to assemble a comprehensive patient-specific montage. 199INTERPRETATION (INTERICTAL) Analysis of the interictal EEG from invasive recordings is an essential prerequisite for proper interpretation of the ensuing ictal data. A methodical approach is advised that reviews the resting record in quiet wakefulness and sleep from all electrodes, with frequent reference to the electrode positions within the brain volume. The baseline amplitude of intracranial EEG is much higher than scalp EEG due to the proximity of intracranial contacts to the generators of EEG and there is a greater presence of fast components. All physiological rhythms recognized on scalp (the waking alpha, frontocentral beta, rolandic mu) are seen on intracranial EEG over the appropriate brain areas (Figure 9.5). The transition to sleep is generally characterized by a progressive appearance of slower rhythms associated with sleep spindles over the rostral brain areas delineating stage N2 sleep (Figure 9.6). However, clear-cut sleep staging is not practicable from intracranial EEG alone, and there is heterogeneity in the response of different cortical regions to sleep–wake state changes. In addition, brain areas “hidden” from scalp recordings exhibit their own characteristic resting rhythms. For example, an abundance of slow (delta) frequencies may be evident over the orbitofrontal cortex even in wakefulness or abundant fast activity over the postero-basal and postero-medial temporal cortex may be present. FIGURE 9.5. Simultaneous appearance of physiological mu rhythm and alpha rhythm in a patient undergoing stereotaxic EEG (SEEG). The implant was undertaken to investigate seizure recurrence after a previous right anterior temporal lobectomy. The implant scheme (inset left) shows the region of previous resection (shaded gray) with orange dots and straight lines indicating planned electrode trajectories. The patient’s left hemisphere was also explored, and is not shown for clarity. Sagittal view of the medial right hemisphere of the preoperative MRI volume with the reconstructed electrodes in situ (inset right) shows the position of the entry of the right posterior insular electrode (RPIN, single arrow) and the medial contacts of the right lingular electrode (RLIN, double arrow). These are also shown on the implant scheme. The entry of the RPIN was through rolandic cortex, anterior to that shown on the implant scheme. The EEG page shows the right-sided SEEG channels in bipolar montage (gain 30 µV/mm, passband 3–70 Hz; gridlines are spaced apart by 1s). Mid-page, the patient is asked to close the eyes and stop moving: the visual alpha rhythm immediately appears (double arrow) as ~10 Hz sinusoidal oscillation maximum in the RLIN5-RLIN6 derivation; simultaneously, the mu rhythm (single arrow) appears over the rolandic area (RPIN11-RPIN12).
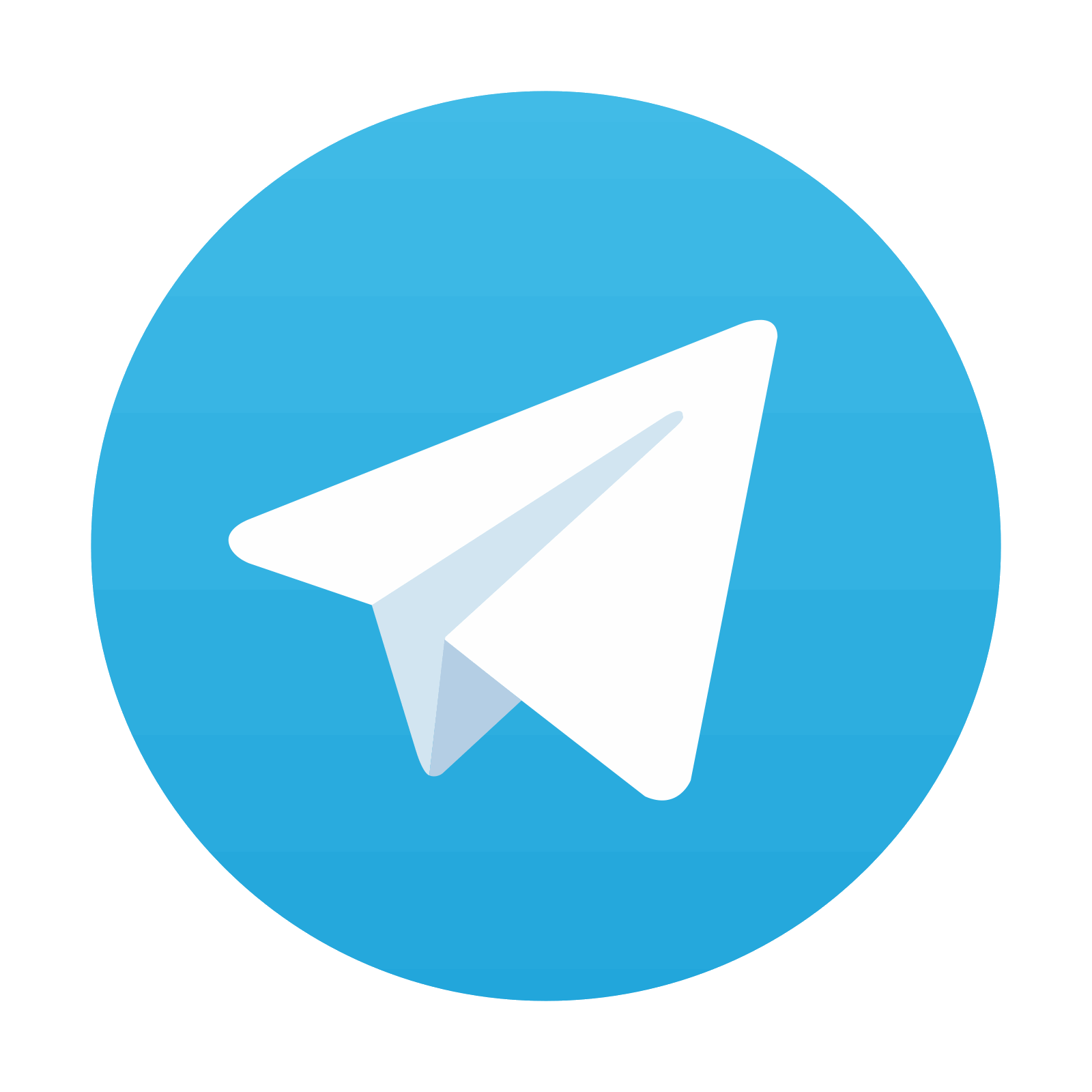
Stay updated, free articles. Join our Telegram channel
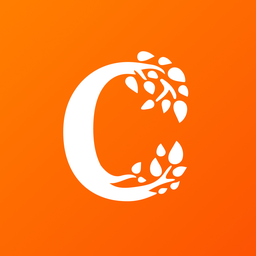
Full access? Get Clinical Tree
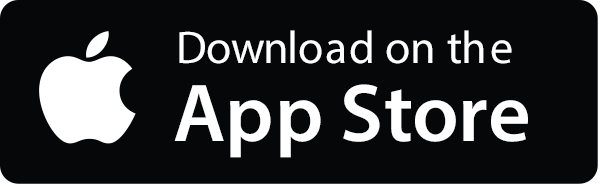
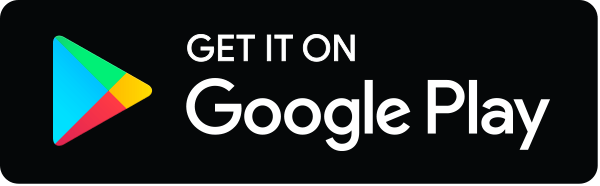