Fig. 1
Common molecular pathways in invertebrates with respect to vertebrates could help in understanding some of the molecular mechanisms involved in autism spectrum disorders. At present more than 500 genes have been related directly or indirectly to ASDs. At least 70 % of these genes have orthologs in the genome of invertebrate animal models. If we imagine vertebrates, like cars, invertebrates can be considered much simpler machines, such as bicycles. In this schema, a “molecular pathway” is represented by a bicycle (a), where each component would correspond to different genes with a specific molecular function. If there is a broken piece, for example, one link in the chain wheel (b) or a flat tire (c), the bicycle does not work. However, if only a tooth on the chain wheel or sprocket is broken (d), then the bicycle works, although occasionally the chain may slip out of place and the functioning of the bicycle can fail more often. When there are other components Fig. 1 (continued) interfering with bicycle movement, for example, disadvantageous environmental factors such as headwind, it is more difficult to make progress with the bike (e). These cases represent a partial loss of function, and the system can undergo a slight reduction in the function of the molecular pathway. Additional damages, for example, other broken tooth on the chain wheel (f) or the interaction between damages and environmental factors (g), can produce a complete loss of function. Thus, invertebrates may facilitate the understanding of the interaction between different genes and environmental factors in pathways implicated in ASDs
Because about at least 70 % of genes related to ASDs have orthologs in the invertebrate animal models, identification of genes as part of a common molecular pathway in invertebrates would help in understanding some disease processes. However, there is an evident difficulty with the invertebrates with respect to ASD research, which raises some questions. To what extent the behavior of a fly or a worm can be translated to what ASD patients are experiencing? What exactly should be evaluated when testing invertebrate mutants for candidate genes involved in ASD? The answers to these questions are not clear, but some behavioral strategies can be envisaged and discussed later in this review.
2 Strategies for Autism Research with Invertebrate Animal Models
In general, two different genetics approaches can be used for carrying out studies of ASD in invertebrates (Fig. 2). The first one is based on reverse genetic strategies, where genes associated to ASDs resulting from large-scale genetic screening in human can be investigated in invertebrate animal models. In reverse genetics, invertebrates might be tested for neurological phenotypes correlating with the disease, such as locomotion, sensorial detection defects, or cellular degeneration [7, 8]. In this respect, genes of interest can be analyzed in four different ways. If an ortholog of the gene under study is present on the genome of the animal model, a knockout strain for this gene can be generated and the effects on phenotype analyzed. It is also of special interest that human genes involved in ASD are functional in the animal model and the mutant phenotype rescued in mutant strains by expression of these specific genes, as is the case of neuroligin and neurexin in C. elegans [9, 10]. Sometimes the knockout is not viable and then alternatively is possible to generate a knockdown organism using RNAi methods, which silence the expression of the gene of interest in a specific period of the life cycle of the animal. RNAi silencing is also helpful when there is more than one ortholog gene, because all of them are suitable to be silenced since they share similar RNA sequence transcripts. Finally, if there is no ortholog on the genome of the model organism, the human disease gene of interest can still be studied by generating transgenic strains which express the human gene in the invertebrate animal. This latter approach proved to be fruitful in studying neurodegenerative disorders, such as Alzheimer’s disease, in Drosophila [8] and C. elegans [11], among others.


Fig. 2
Genetics approaches for research in autism spectrum disorders with invertebrate animal models. Two genetic strategies can be applied for studies of ASD in invertebrates. Reverse genetics uses the information of genes associated to ASD in human by obtaining knockout and knockdown or/and by expression of human genes. Rescue experiments expressing human genes in knockouts or overexpressing them in knockdown animals can confirm the functionality of the human genes in the model organism. Forward-genetic screening, using random mutations in the genome, and a screening with a library of RNAi are useful methods to identify other genes involved in molecular pathways of biological processes implicated in ASDs. In reverse- and forward-genetic strategies, it is necessary to develop phenotypic assays. These two strategies can be combined with the study of the effects of drugs, hormones, or some environmental factors that have been related to ASDs
A second strategy could be used based on forward-genetic screening, using random mutations in the genome induced by a mutagen such as ethyl methanesulfonate [12] or a screening with a library of RNAi [13]. In both cases it is necessary to have developed a phenotypic assay to test specific phenotypes. Both are useful methods to identify genes involved in molecular pathways of biological processes implicated in specific behavioral phenotypes. For example, forward-genetic screens have played a key role in detecting genes that control sleep in Drosophila [14].
These two strategies can be combined with the study of the effects of drugs, hormones, or some environmental factors that have been associated to ASDs on behavioral phenotypes of the model animal. For example, we observed that methylphenidate and fluoxetine can restore behavioral impairments related to dopamine and serotonin in nlg–1-deficient mutants of C. elegans [156] or that testosterone, proposed to be linked to ASD phenotype [15], is able to modify some behaviors of wild-type C. elegans worm [157].
3 Drosophila melanogaster
Drosophila is an important model for genetics disease studies. The fly has a relatively simple genome with about 139 million base pairs and approximately 16,000–17,000 genes distributed on four pairs of chromosomes [16]. The fly brain consists of approximately 100,000 neurons [17], much smaller than the 100 billion neurons in the human brain. It is estimated that 75 % of human genes that have been related to human diseases are represented in the Drosophila genome [18]. Although the fruit fly and human lineages diverged a few hundred million years ago, they share many basic cellular and neurobiological pathways.
There are several characteristics that make Drosophila an established and profitable tool for studying ASDs and other complex neurodevelopmental and behavioral disorders [19–21]. The main features are the complex behavioral range of abilities of the fly to adapt to environmental factors and experiences, the short generation time, the relatively low cost for handling in the laboratory, as well as the possibility of applying an enormous battery of genetic and molecular biology methodologies, including transgenic techniques [22].
Given the different physiologies and cognitive abilities of flies and humans, many behavioral features of the human disorders cannot be studied in flies, but in spite of this dissimilarity, Drosophila is progressively more used for studying basic mechanisms, including neurodegenerative and neurological diseases, such as Parkinson’s disease, Alzheimer’s disease, or fragile X syndrome. Furthermore, a growing understanding of the genetics of human neuropsychiatric disorders is suggesting analogous neurobiological mechanisms between human and flies, and this tiny model organism is providing significant clues for understanding the etiology of complex psychiatric disorders [19].
Drosophila has a wide repertoire of behaviors that can be studied and analyzed relatively easily in the laboratory. Among them are reactions to several sensory stimuli including attraction or repulsion to odors and noxious substances, and locomotor responses to movement of objects and vibrational stimuli [23]. In addition, the capacity for learning and memory has been confirmed in the fly. Perhaps one of the best studied examples is olfactory associative learning, in which larvae learn to associate specific odors with either a punishment or a reward [24]. The memory can be separated from short-term memory within seconds or minutes to long-term memory that lasts for days [25–27]. Drosophila is also a good model for studying mechanisms of sleep [28–30]. Sleep disturbances are symptoms that might be associated with several neuropsychiatric disorders, including ASDs [31, 32]. Although Drosophila cannot be considered a social animal, flies show a variety of social behaviors ranging from simple aggregation [33] to complex conducts like courtship [34–36] and aggression [37]. These behaviors appear plastic to some degree and are suitable to be analyzed in the complex neuronal circuits of the fruit fly [38, 39].
Autistic symptoms are often present in some heritable neurodevelopmental disorder conditions that can be associated to intellectual disability, cognitive dysfunction, and other behavioral impairments. Most of these disorders have a multifactorial origin, but some of them are monogenetic, as is the case of Rett syndrome and fragile X syndrome [40]. The deep knowledge in the Drosophila genetic system has provided advances in the understanding of the molecular basis of ASD taking advantage of these monogenic disorders [41].
3.1 Rett Syndrome
The Rett syndrome is an important cause of female intellectual disability in girls. They normally have a typical development until 6–18 months, and then it is followed by a loss of acquired skills in motor abilities, cognitive impairment, autistic behaviors, and seizures [42]. In 90 % of Rett cases, the origins are mutations in the gene MECP2, located in the X chromosome (Xq28). MECP2 mutations have been recognized in idiopathic ASD patients [43]. MECP2 encodes methyl CpG-binding protein 2, a widely expressed transcriptional repressor of methylated DNA [44]. Increased dosage of the MECP2 gene also leads to Rett-like features and mental retardation [45, 46]. Drosophila does not have a MECP2 gene ortholog in its genome, and therefore mutations or knockout cannot be generated and analyzed. However, human MECP2 gene has been overexpressed in the fly. In these transgenic flies, the MeCP2 protein was found to be associated with chromatin in Drosophila, as it was described in mammals, and moreover unexpectedly it was also phosphorylated at serine 423 [47]. The overexpression of MECP2 in Drosophila produced some anatomical deficiencies, such as disorganized eyes, and behavioral defects in a climbing assay, which suggested a motor dysfunction [48].
3.2 Fragile X Syndrome
Drosophila is a good model for fragile X syndrome (FXS), a condition clinically characterized by delayed developmental symptoms, memory deficits, sleep disorder, hypersensitivity to sensory stimuli, seizures, elevated anxiety, and hyperactivity [49]. Furthermore, approximately 30 % of those with FXS meet the diagnostic criteria for ASD [50, 51]. The cause is almost always an unstable CGG-trinucleotide repeat expansion in the regulatory region of the FMR1 gene [52, 53]. This expansion causes hypermethylation and transcriptional silencing of FMR1, resulting in loss of its gene product, the protein FMRP (fragile X mental retardation protein). The FMRP is an mRNA-binding protein involved in transcript stability, trafficking, and translation control. Subsequent molecular advances showed that FMRP regulates the translation of many genes involved in the neuronal synapse [54, 55]. The fruit fly has a single FMR1 gene ortholog, named dfmr1. The deletion of dfmr1 in Drosophila produces some phenotypes that remind some associated with FXS in human. FMRP overexpression or deletion and its effect in Drosophila have been recently reviewed [41].
In respect to ASD, social interaction has been assayed in mutants defective in dfmr1. The dfmr1 knockout was hypoactive and showed less interaction with other flies and scored a decrease in the sociability index. But interestingly, the dfmr1-defective mutants interact more with wild-type flies than with other dfmr1 mutants [56]. This observation suggested that Drosophila requires an interactive response to maintain engagement with a partner. This is in some respect similar to the response to interaction exhibited in a form of motor dyspraxia, often described in ASD, where motor coordination can affect planning of movements and coordination, probably as a result of brain messages not being correctly transmitted [57–59].
3.3 Cell Adhesion Molecules: Neurexin and Neuroligin
The development of the nervous system requires that each neuron finds and makes synapses with other neurons correctly. Neurexins and neuroligins are two cell adhesion molecules identified as central organizing molecules for excitatory and inhibitory synapses [60–63]. They have been implicated in various neuronal processes, including the differentiation, maturation, stabilization, and plasticity of synapses [64]. Neuroligins alone can induce the formation of fully functional presynaptic terminals in contacting axons [65, 66]. On the other hand, neurexins alone can induce postsynaptic differentiation and clustering of receptors in dendrites [67]. The importance of the genes encoding neurexin and neuroligin is highlighted by the observation that mutations in these genes in humans have been associated with autism spectrum disorders and other psychiatric conditions [68, 69]. In Drosophila neurexin and neuroligin also play a crucial role in behavioral and cellular processes [70, 71]
In mammals, neurexin seems to nucleate the aggregation of components belonging to a synaptic cell adhesion complex. Thus, neurexin has been shown to bind to several key synaptic proteins, including the vesicle protein synaptotagmin [72], the scaffolding proteins Mint [73] and CASK [74], and the cell adhesion molecules neuroligin [75] and LRRTM2 [76]. Recently it has been found that alternative splicing of presynaptic neurexin-3 controls postsynaptic AMPA receptor trafficking [77].
Drosophila appears to have only a single neurexin-encoding gene, similar to mammalian alpha neurexin [78]. The honeybee has also only one gene encoding neurexin, but in this insect alternative isoforms have been reported by differential splicing of 12 splice sites, and some of these variants are similar to vertebrate alpha- and beta-neurexin isoforms [79]. Neurexin knockout Drosophila is viable and fertile but their nervous system has a smaller number of synapses and shows an impaired synaptic transmission. In embryos neurexin is present both pre- and postsynaptically, promoting active zone formation and neurotransmitter release in the presynaptic membrane and suppressing the formation of ectopic glutamate receptor clusters in postsynaptic membrane [80]. Neurexin was reported to be important for locomotion through interaction with scaffold Drosophila protein (CAKI/CMG), a homologue of vertebrate CASK [81]. In addition, neurexin-defective mutant larvae have defects in associative learning behavior [78, 82]. Figure 3a shows a scheme of the experiments in relation to larva associative learning performance in wild type compared to the neurexin knockout mutant strain. In these experiments reciprocal training of two groups of Drosophila larvae was performed. One group was exposed to n-amyl acetate (AM) with a sugar reward and then subsequently exposed to 1-octanol (OCT) with no reward. The other group received the reciprocal training. The two groups of larvae were then tested for their choices between AM and OCT. Preferences for AM or OCT depending on previous experiences with food location revealed an associative learning behavior [82].


Fig. 3
Phenotypes related to autism spectrum disorders in neuroligin and neurexin mutants of Drosophila melanogaster. (a) Neurexin-deficient mutant (dnrx-def). Larvae need to eat continuously and therefore food represents a powerful reward. A group of larvae is placed onto a sweet-tasting sugar substrate (+) presented with a specific odor A (denoted as A+). After this exposure, the larvae are transferred to a sugar-free situation, characterized by another odor (denoted as B−). Conversely, the experiment is done the other way around, sugar was put in the presence of B (denoted as B+) instead of A (denoted as A−). After three such A+/B− experiences or A−/B+, the larvae are tested by offering them a choice between the two odors in the absence of sugar. If they have associated odor with the reward, they should track down this odor in search of sugar. Wild-type larvae exhibit associate learning behavior, and they move to the odor where the reward, the sugar, was placed at the beginning of each experiment. The neurexin-defective mutants (dnrx-def) of Drosophila are impaired in associative learning behavior. See text for details and references. (b) Neuroligin-deficient mutant (dnl2-def). (a) Courtship: Extension of one wing by wild-type males is limited to short periods of courtship in the immediate surrounding area of a female. Dnl2-deficient mutant does not finish courtship posture retracting the wing and keeps it in the extended position for significantly prolonged periods of time. (b) Courtship song: Acoustic communication signals of dnl2-deficient male are impaired in relation to wild type. These mutants have shorter inter-pulse intervals of duration than wild type. Sine song intensity is also reduced in dnl2-deficient mutant Fig. 3 (continued) males compared to wild-type ones. (c) Aggressiveness: When aggressive encounters were initiated in mixed genotype assays with wild-type and dnl2-deficient strains, the duration of aggressiveness behavior was significantly prolonged in wild-type males in relation to dnl2-deficient ones. Dnl2-deficient males initiated courtship as frequently as wild types in equal genotype assays but displayed reduced courtship initiation in direct competition with wild-type males. Based on data published by Hahn et al. [91]
There are several observations in which truncating mutations in neuroligin genes were associated with ASDs and/or intellectual incapacity [83–86]. As in humans, some insects including honeybee (Apis mellifera) and mosquito (Anopheles gambiae) have five neuroligin genes [79], but Drosophila genome has only four neuroligin genes (dnl1–4). The dnl1 gene specifically is expressed in muscle and dnl2 gene is expressed in both muscle and postsynaptic neuronal membranes [87–90]. Both genes dnl1 and dnl2 have been studied in the formation and function of neuromuscular junctions. The absence of either dnl1 or dnl2 does not avoid formation of the neuromuscular junction, but the deletion of dnl1 reduced synaptic excitation [87], and the deletion of dnl2 increased it [90]. The absence of dnl2 gene also impairs social interactions, altering acoustic communication signals, performing less female-directed courtship and male-directed aggressive behaviors without changing the patterns of locomotor activity [91]. This suggests that the reduced social interactions may result from altered excitability in the central nervous system. On the other hand, since olfactory, visual, and auditory perception tests revealed no sensory impairments of deficient mutants in dnl2, Hahn et al. (2013) have concluded that neuroligins are phylogenetically conserved and are involved with the regulation of social behaviors in common ancestors of humans and flies. Figure 3b shows a summary of the impaired social behaviors, including courtship wing movement, courtship song, and aggressiveness indicator, found in defective dnl2 fruit fly compared to wild-type strain.
4 Aplysia californica
Aplysia californica is a species of gastropod mollusk, a large sea slug. Aplysia has a small number of central nervous system neurons, 20,000, compared to the 100,000 of Drosophila and the 1,000,000,000,000 of mammals; although it has an enormous number of neurons compared to C. elegans, which has only 302 in the hermaphrodite and 381 in the male. A. californica, as all the sea slugs, is hermaphroditic, acting as male and female simultaneously during mating. In Aplysia the size of neurons is an advantage, which are of the largest somatic cells known in the animal kingdom having a diameter ranging from 0.1 to 1 mm.
Aplysia neurons are so large that antibodies, chemicals, and DNA can relatively easily be injected into them [92] and cDNA libraries can be made out of individual cells [93]. Also, in this slug there are small groups of neurons directly related to individual behaviors, making the study of learning, memory, and social behavior much more feasible. The neurons can also be cultured in vitro and they are excellent tools for the study of synaptogenesis and neural development [94].
The Aplysia gill-withdrawal reflex (GWR) is an involuntary defense to tactile stimulation (Fig. 4a). The study of mechanisms of GWR has been essential for establishing the biochemical and neuroanatomical basis of learning and memory [95–97]. In addition, Aplysia has helped in understanding the plasticity of the nervous system, and the environment can exert a structural and functional effect on the nervous system. Experiments with Aplysia demonstrated that learning and memory are mediated by pre- and postsynaptic mechanisms [98]. Memory can occur as short term and long term. In the short-term memory, there is an increase in the efficiency by which nerve impulses pass across synapses. It does not require any protein synthesis or remodeling of the synapse. However, the long-term memory lasts for a long period and requires gene expression and protein synthesis and involves the formation of new synaptic connections [99].


Fig. 4
Aplysia californica as a model to study autism spectrum disorder. (a) The gill-withdrawal reflex (GWR). Many experiments in Aplysia are based on the GWR. The GWR is an involuntary defensive reflex. The gill is used for breathing, and it can be extended or retracted and also can be covered with the mantle shelf to protect it. Wastes and seawater are released through the siphon. The siphon has 24 sensory neurons with touch receptors. The cell bodies of these neurons are located in the abdominal ganglion, a small group of neuronal cell bodies, which send out dendrites to pick up environmental information to the siphon. After collecting sensory information, they pass it on to other neurons throughout their axons. Siphon sensory neurons form synapses with excitatory and inhibitory interneurons that connect with motor neurons that connect with muscular cells of the gill. So if siphon is touched, it sends signals via the sensory neurons in the abdominal ganglion to motor neurons in the gill, making it to retract and originating the gill-withdrawal reflex. (b) Habituation and sensitization. Aplysia shows several types of behavioral plasticity; among them are habituation and sensitization. Habituation: when applying a tactile stimulus to the siphon, withdrawal of the gill results, but repeated siphon stimulation causes the withdrawal to decline. Sensitization: when there is habituation to siphon touching, applying a harmful electrical stimulus to the tail paired with a light touch of the siphon elicits a strong withdrawal, as if the animal had not been habituated. These reflexes can be divided in short- and long-term memory. If Aplysia receives a single stimulus to the tail, the gill-withdrawal reflex remains enhanced for about an hour (short-term memory). When repeating pairing of tail and siphon stimuli, the sensitization can be maintained for weeks (long-term memory). Hundreds of neurons are involved in gill withdrawal, but during habitation and sensitization, only a few different types of neurons are implicated. The key neurons are sensory neurons that innervate the siphon and tail, motor neurons that innervate muscles in the gill, and interneurons that receive inputs from the sensory neurons. See text for more details
Aplysia show several types of behavioral plasticity like habituation and sensitization (Fig. 4b). Habituation is a reduction in a previously displayed response when no reward or punishment follows. After touching the siphon once, a gill-withdrawal reflex occurs producing a retraction of the gill. If the stimulus is given repeatedly and nothing either pleasant or unpleasant happens, the gill-withdrawal reflex will soon cease to respond. This lack of response is not a result of fatigue or sensory adaptation and is long lasting; when fully habituated, the slug will not respond to the stimulus even though weeks have elapsed since it was last presented. It has been shown that habituation of withdrawal requires protein synthesis, protein phosphatase activity, and activation of NMDA-type and AMPA-type postsynaptic receptors within the abdominal ganglion [100].
Sensitization is an increase in the response to an innocuous stimulus when that stimulus occurs after a noxious or punishing stimulus (Fig. 4). For example, when the siphon of Aplysia is gently touched, the animal withdraws its gill for a brief period of time. However, if preceded by an electrical shock to its tail, the same gentle touch to the siphon will elicit a longer period of withdrawal. The sensitization response to a single shock finishes after about an hour and returns to the baseline after a day. This is an example of short-term memory. The biochemical mechanisms responsible for the enhancement of glutamatergic transmission during short-term sensitization could be due to serotonin, which released by interneurons binds to G-protein-coupled receptors (GPCR) in the cell body and terminals of the sensory neuron in the siphon-gill pathway. The GPCR initiates the production of adenylyl cyclase, which catalyzes the formation of the second messenger cAMP [101]. The rise in cAMP activates a cAMP-dependent protein kinase (PKA) which phosphorylates several proteins, including K+ channels. When a sensory neuron is depolarized, Ca2+ channels open, and Ca2+ enters the neuron. PKA phosphorylates K+ channels resulting in fewer K+ pumping out, prolonging the duration of the depolarization and increasing the influx of Ca2+, which increases the release of glutamate [102, 103]. Therefore this process enhances synaptic transmission between the sensory and motor neurons within the gill-withdrawal circuit.
The same serotonin-induced enhancement of glutamate release that mediates short-term sensitization is also thought to be involved in long-term sensitization, prolonged for up to several weeks. This form of plasticity is due to changes in gene expression and protein synthesis. It is suggested that with repeating tail shocks, the serotonin-activated PKA now also phosphorylates the transcriptional activator cAMP-response element-binding protein (CREB) [104]. The activated CREB binds to cAMP-response elements (CREs) in regulatory regions of nuclear DNA, increasing the rate of transcription of specific genes, which augment the synthesis of ubiquitin hydrolase and protein C/EBP. Ubiquitin hydrolase is involved in the degradation of the regulatory subunit of PKA that causes a persistent increase in the amount of catalytic subunit, and then PKA does not need serotonin to be activated. C/EBP is also a transcription factor that is involved in the transcription of genes implicated in the formation of new synaptic terminals [99]. As a result of this process, a long-term sensitization is established by increasing the number of synapses between the sensory and the motor neurons.
Transcriptional regulation is considered the initial point for a sequence of molecular steps needed for both the initiation and maintenance of long-term synaptic facilitation. Gene regulation by CREB acting in combination with different transcriptional factors is decisive for the expression of many forms of long-term memory [105]. The essential molecular characteristics of transcriptional regulation involved in the long-term process are evolutionally conserved in Aplysia, Drosophila, and mouse, and it is thought that similar mechanisms may underlie the synaptic changes that account for long-term memories in humans [106].
The role of CREB seems to be also conserved in the little nematode C. elegans (see next section). In relation to this, the response to tap, a kind of nonlocalized mechanosensory stimulation, and tap habituation has been investigated. Worms with a loss-of-function mutation in crh-1, encoding a CREB ortholog, are impaired in reversals to tap and did not show long-term memory for spaced training 24-h post-training; however, they did show short-term habituation to tap when stimuli were presented at both 10-s and 60-s interstimulus intervals and also showed 12-h intermediate memory for spaced habituation training. Thus, CREB seems to be required for long-term habituation in C. elegans [107]. Other experiments of aversive olfactory learning and associative long-term memory have confirmed that like Aplysia, Drosophila, and mice, the worm needs CREB for long-term memory and not for short-term or middle-term memory [108, 109].
The role of neurexin and neuroligin has been investigated at sensory-to-motor neuron synapses of GWR in Aplysia. Depleting neurexin in the presynaptic sensory neuron or neuroligin in the postsynaptic motor neuron was found to eliminate synapse facilitation. Moreover, introduction into the motor neuron of the R451C mutation of neuroligin-3, which was linked to ASD, blocked both intermediate-term and long-term synapse facilitation and also the presynaptic growth originated by repeated pulses of serotonin. These results suggest that activity-dependent regulation of the neurexin-neuroligin interaction may govern transsynaptic signaling required for the storage of long-term memory but also emotional memory that is impaired in ASD [110].
5 Caenorhabditis elegans
The difficulties in the advance in the knowledge of neurodevelopmental disorders using mammals as animal models are in part because their wiring maps are highly complex. Many invertebrates have an intricate nervous system. For example, the nervous system of Drosophila has around 100,000 neurons and is only partially characterized [17, 39, 111–113]. C. elegans is a well-established model organism that has some exceptional characteristics for studying behavior and neurological diseases [4, 114–116]; its nervous system consists of 302 neurons [117], being their pattern of synaptic connectivity well characterized [118]. It has been estimated that over 83 % of the nematode proteome has human orthologs proteins [119], and that is a significant factor for using it as a model to decipher molecular mechanisms and pathways involved in complex disorders in human.
The worm uses the classical neurotransmitters such as serotonin, acetylcholine, dopamine, glutamate, and GABA, although epinephrine, norepinephrine, and histamine seem to be absent [120]. In addition, other basic synaptic components of the nematode are similar to the mammalian nervous system, among them ion channels, vesicular and neurotransmitter transporters, receptors, adhesion molecules, and components of the postsynaptic density (Fig. 5b) [121, 122]. These facts make the worm a useful tool for understanding synaptopathies and action mechanisms of drugs used in psychiatric disorders [123, 124]. Thus, C. elegans nervous system presents molecular targets to test human drugs such as fluoxetine and imipramine (antidepressants), cocaine and nicotine (alkaloid drug), or ethanol (psychoactive drug) [125]. Other chemicals such as the antiparasitic dugs aldicarb and levamisole, inhibitor of acetylcholinesterase and agonist of acetylcholine, respectively, are widely used to study pre- and postsynaptic impairment [10, 126].


Fig. 5
Phenotypes related to autism spectrum disorders in neuroligin and neurexin mutants of Caenorhabditis elegans. (a) Characteristic phenotypes of nrx-1– and nlg-1-deficient mutants. U pper panel, the exploratory behavior in C. elegans is measured as the fraction of the area on the plate explored by the animal (dotted lines). Neuroligin-deficient mutants show a hyperactive behavior with respect to wild-type animals; neurexin-deficient mutants have severe deficits in exploratory capacity. Central panel, C. elegans respond with an aversive manner to high osmotic pressure—a 4 M fructose solution (represented by a gray ring into the plate). Neuroligin-deficient mutants are unable to detect this solution and they do not come back when they encounter the 4 M fructose barrier. Lower panel, the nematode left sinusoidal tracks on a bacterial lawn (shown as gray patch). The wavelength and amplitude of the weaves can be measured. The neurexin- and neuroligin-deficient mutants show differences in both wavelength and amplitude of their tracks, compared with wild-type animals. (b) Representative scheme of a glutamatergic synapse in C. elegans. Neurexin (NRX-1) and neuroligin (NLG-1) are located at the pre- and postsynaptic membranes, respectively. NRX-1, through its intracellular domain, could bind to structural proteins in the presynaptic terminal such as PDZ, LIN-2, and LIN-10. Also, in the presynaptic terminal, synaptobrevin and syntaxin (SBN-1 and UNC-18) along with the SNARE complex lead to vesicle fusion and neurotransmitter release to the synaptic cleft. In a similar manner, in the postsynaptic site, some protein interactors join the intracellular domain of NGL-1 and neurotransmitter receptors. (c) Epifluorescence image (upper) and merged fluorescence-Nomarski image (lower) from the BC13247 transgenic strain. This strains harbor a transcriptional construction, expressing the GFP protein under the nlg-1 promoter. The images show cell body neurons expressing GFP in head ganglia and around nerve ring. Scale bar corresponding with 15 μm
Classical and molecular genetics, large-scale mutagenesis screens, gene expression studies, genomic analysis, and generation of transgenic animals are perhaps more easily performed and less time consuming in C. elegans than in most other experimental animal species. Another useful methodology to analyze the function of a specific neuron in the worm is the application of laser ablation of a particular neuron or group of them, since the nematode required only a few cell types for its viability [127–129]. The elimination of cells and the subsequent behavioral abnormalities in the nematode provide a powerful knowledge concerning in vivo neuronal function [130]. The worm is also amenable to high-throughput analysis with specific flow cytometer technologies [131]. Taking advantage of the transparency of this nematode, there are fluorescent markers for practically the majority of neurons allowing the study in vivo of the synaptic function in specific neurons in a very accurate manner (Fig. 5c) [114].
Together with its relatively short life span, low costs in growing, fast reproduction, and high progeny number, perhaps one of the more helpful tools in the nematode is the possibility to generate knockdown animals with RNAi by feeding [132]. In C. elegans the large-scale genetic screenings are used extensively through generation of knockdown animals. Worms can be grown on agar plates where they feed on bacteria that produce dsRNA, which is processed into siRNA by the nematode, downregulating the expression of specific target genes [133]. In this respect some neurons are resistant to the silencing RNAi effect [134], but it was established that a genetics background with a defective rrf-3 gene, which encodes a putative RNA-directed RNA polymerase, produces an increase to RNAi sensitivity in most neurons [135]. Also, the expression of sid-1, encoding a transmembrane protein required for systemic RNA interference in C. elegans, increases the sensibility of neurons to dsRNA provided by feeding [136].
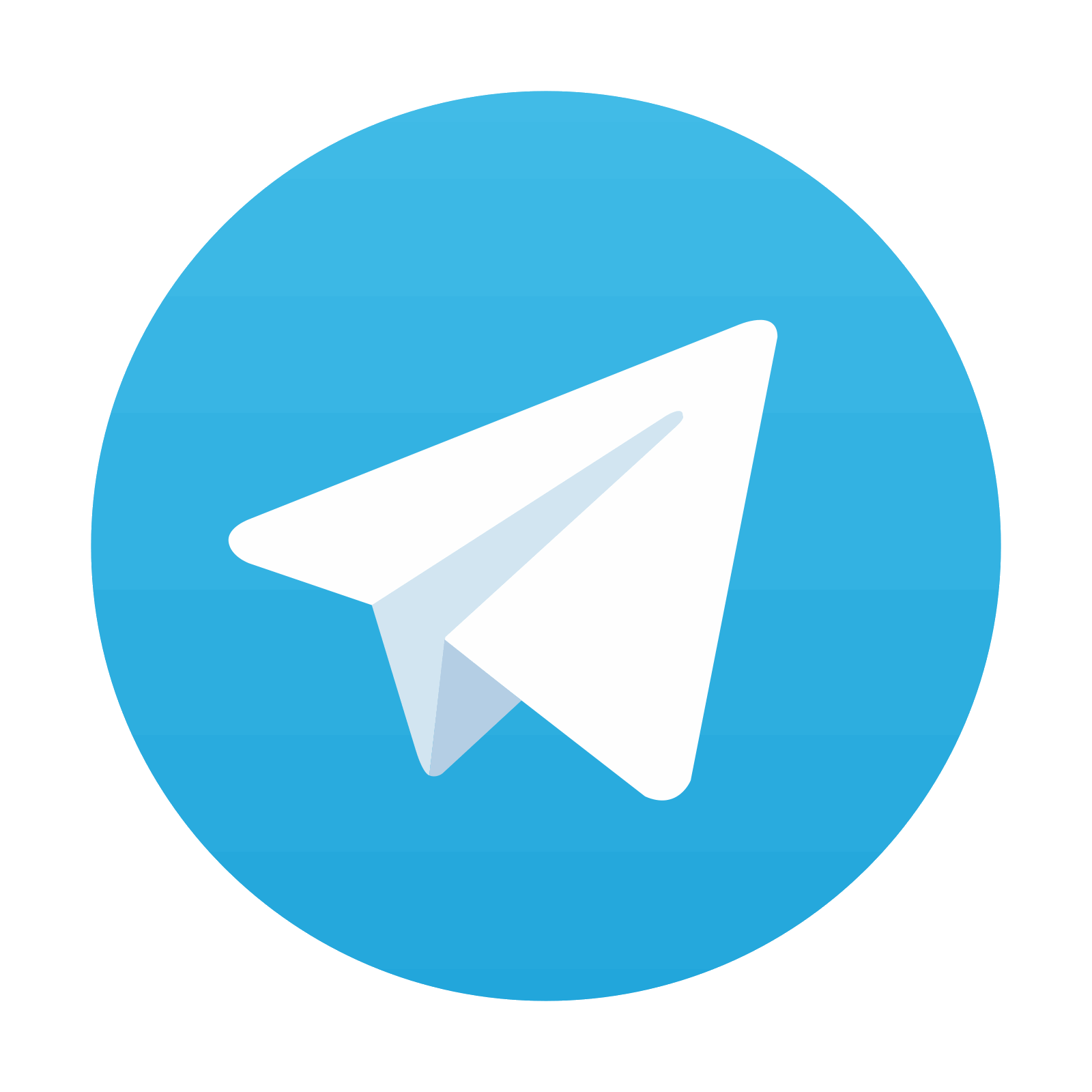
Stay updated, free articles. Join our Telegram channel
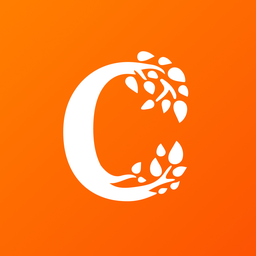
Full access? Get Clinical Tree
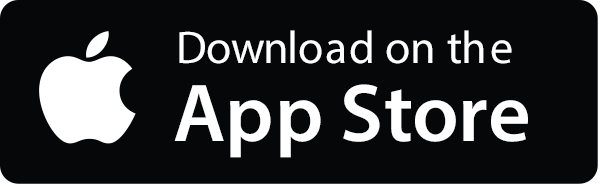
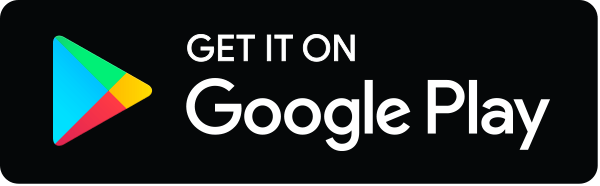