Objectives
- 1.
Compare and contrast the properties of voltage-gated Ca 2+ and Na + channels.
- 2.
Describe the mechanism of action of Ca 2+ antagonist drugs, and describe their use as therapeutic agents.
- 3.
Describe the properties of TRP channels.
- 4.
Describe the role of A-type K + channels and Ca 2+ -activated K + channels in regulating the AP firing pattern in a bursting neuron.
- 5.
Describe the properties of ATP-sensitive K + channels, and explain their role in glucose-induced insulin release from pancreatic β-cells.
- 6.
Describe the role of hERG K + channels in repolarizing the cardiac AP.
- 7.
Explain how the activation of β-adrenergic receptors modulates Ca 2+ channel activity and how this modulation enhances force development in the heart.
Various types of ion channels help regulate cellular processes
Ion channels are found in all cells. The properties of voltage-gated Na + and K + channels, which are essential for generating the AP in nerve axons and skeletal muscle cells, are described in Chapter 7 . Many other types of ion channels also play important roles in regulating membrane electrical activity. Table 8.1 lists some examples. Each of the ion channel types is defined by a combination of specific physiological, pharmacological, and structural characteristics. As an example, the characteristics of voltage-gated Na + channels in nerve axons include, in part, Na + selectivity, voltage-dependent activation, fast inactivation, and high-affinity block by tetrodotoxin. In this chapter we focus on the properties of several other physiologically important channels. These examples illustrate the diversity of ion channel types, the variety of roles they play in normal cell function, and their pathophysiology. Ion channels that open after the binding of a neurotransmitter or other chemical activator, called ligand-gated ion channels, are discussed in Chapters 12 and 13 .
Channel Type | Location | Functions |
---|---|---|
Voltage-Gated Channels | ||
Na + channel | Axon, skeletal muscle | Upstroke of the AP |
K + channels | Axon, skeletal muscle | AP repolarization |
Ca 2+ channels (many types exist) | Heart, nerve terminals, endocrine cells | Inject Ca 2+ into cells |
Ligand-Gated Channels | ||
Nicotinic ACh receptor channel | Neuromuscular junction, neurons | Synaptic transmission |
Glutamate receptor channels | Neurons | Synaptic transmission |
GABA receptor channel | Neurons | Synaptic transmission |
Ca 2+ -activated K + channels | Almost all excitable cells | Attenuate electrical excitability |
Other Channels | ||
ATP-sensitive K + channel | Pancreas, heart, smooth muscle | Glucose sensor in β-cells |
Inward-rectifier K + channel | Heart, brain, skeletal muscle | Permit long depolarizations |
Store-operated channels | Nearly all cells | Regulation of [Ca 2+ ] i |
Receptor-operated channels | Nearly all cells | Regulation of [Ca 2+ ] i |
Two-pore K + channel | All cells | Regulate resting potential |
CRAC Channel (ORAI) | Lymphocytes, platelets, muscle, other cells | Store-operated Ca 2+ entry |
SUR1-TRPM4 a | Astrocytes | Cytotoxic edema |
a The channel is a heteromeric coassembly of sulfonylurea receptor 1 (SUR1) protein and TRPM4 subunits.
Voltage-gated Ca 2+ channels contribute to electrical activity and mediate Ca 2+ entry into cells
Ion channels that selectively allow Ca 2+ ions to permeate are of vital importance in the normal functioning of nearly all cells. All excitable cells have Ca 2+ channels. In some cells, voltage-gated Ca 2+ channels can generate APs in the absence of Na + channels. However, in many cells that express both voltage-gated Na + and Ca 2+ channels, Ca 2+ channel activity modifies the shape of the AP. Ca 2+ channels contribute to the APs generated in the heart ( Fig. 8.1 ), some endocrine cells (e.g., pancreatic β-cells), and specific regions of neurons (e.g., dendrites and nerve terminals).

Ca 2+ channels influence a wide range of cellular activities by allowing Ca 2+ ions to enter the cell. The extracellular free Ca 2+ concentration is approximately 1 mM (10 −3 M), whereas [Ca 2+ ] i is normally approximately 0.0001 mM (10 −7 M). This means that E Ca is approximately +120 mV. Therefore, when a Ca 2+ channel opens, Ca 2+ ions flow into the cell down their electrochemical gradient. Because [Ca 2+ ] i is normally very low, a relatively small influx of Ca 2+ can produce a significant increase in [Ca 2+ ] i . [Ca 2+ ] i directly regulates secretion, contraction, energy metabolism, ion channel activity, and numerous other processes.
Several distinct types of voltage-gated Ca 2+ channels have been identified based on their physiological, pharmacological, and structural properties ( Box 8.1 and Table 8.2 ). Indeed, several Ca 2+ channel mutations have been identified that are associated with hereditary diseases. One example is X-linked congenital stationary night blindness. This is caused by loss-of-function mutations in the L-type Ca 2+ channel subtype that is expressed in photoreceptors ( Box 8.2 ).
Multiple types of voltage-gated Ca 2+ channels have been identified on the basis of physiological, pharmacological, and structural criteria. These include the following.
L type (Ca v 1)
The L type is the major type of Ca 2+ channel in muscle cells (cardiac, smooth, and skeletal). L-type Ca 2+ current is characterized by a relatively high voltage for activation, fast deactivation, slow voltage-dependent inactivation, a large single-channel conductance, modulation by cAMP-dependent phosphorylation, and inhibition by dihydropyridines, phenylalkylamines, and benzothiazepines. These Ca 2+ channels are called L-type channels because of their l arge conductance (25 pS) and l ong-lasting single-channel open times.
T type (Ca v 3)
In comparison with L-type Ca 2+ currents, T-type currents activate at more negative voltages, have fast voltage-dependent inactivation, have slow deactivation, have a small single-channel conductance (8 pS), and are insensitive to the drugs that block L-type channels. They are named T type for their t iny single-channel conductance and their t ransient kinetics.
N type (Ca v 2.2)
N-type Ca 2+ currents were first identified by their intermediate voltage-dependence and intermediate inactivation kinetics. The channels are insensitive to the L-type Ca 2+ channel blockers but are blocked selectively by a cone snail peptide toxin, ω-conotoxin GVIA. They were called N type because they were n either L type n or T type.
P/Q type (Ca v 2.1)
P-type Ca 2+ currents were first recorded in cerebellar Purkinje neurons and are blocked selectively by the spider toxin, ω-agatoxin IVA. Q-type Ca 2+ currents were first recorded in cerebellar granule cells and have a lower affinity for ω-agatoxin IVA.
R type (Ca v 2.3)
R-type Ca 2+ currents are activated by high voltage but are insensitive to dihydropyridines, ω-conotoxin, or ω-agatoxin IVA and are therefore referred to as “residual” or R-type. They are thought to play a role in neurotransmitter release. The channels are blocked selectively by SNX-482, a peptide toxin found in tarantula venom.
X-linked congenital stationary night blindness (CSNB) is a recessive retinal abnormality characterized by night blindness, decreased visual acuity, and myopia. Two distinct clinical types are observed, and they exhibit different photoreceptor (rod and cone) abnormalities. With complete CSNB the rods are completely nonfunctional and the cones are normal. With incomplete CSNB, function of both rods and cones is depressed but measurable. The disorder is caused by mutations in retina-specific L-type Ca 2+ channels (different isoforms of the L-type Ca 2+ channel are expressed in different cell types). In incomplete CSNB, mutations in the L-type Ca 2+ channels in both rods and cones result in reduced activity of Ca 2+ channels. The consequent reduction in Ca 2+ entry decreases the tonic release of the neurotransmitter glutamate from photoreceptor nerve terminals. As a result, synaptic transmission from the photoreceptors to the second-order bipolar cells is inhibited. The bipolar cell is an interneuron that transmits information from photoreceptors to ganglion cells, and axons of the ganglion cells form the optic nerve. Thus the reduction in glutamate release from photoreceptors results in decreased output from the optic nerve, which helps explain the visual defects that characterize CSNB.
Ca 2+ currents can be recorded with a voltage clamp
Voltage-gated Ca 2+ channels can be studied with the voltage clamp. Whole-cell currents recorded from a neuronal cell body with a voltage clamp are shown in Fig. 8.2 . With K + channels blocked, a depolarizing voltage clamp step evokes an inward current that has both a fast transient component and a more sustained component. The fast transient component of the current is generated by Na + channels. When external Na + is removed, the Na + channels no longer conduct inward current (because there are no Na + ions to flow into the cell), and the remaining inward current is carried by Ca 2+ ions flowing into the cell through voltage-gated Ca 2+ channels ( Fig. 8.2 ). The Ca 2+ channels activate more slowly than Na + channels. Furthermore, the magnitude of the Ca 2+ current ( I Ca ) decreases very slowly over tens of milliseconds. This indicates that Ca 2+ channels inactivate slowly (much more slowly than Na + channels). Inactivation also is incomplete, so that Ca 2+ current can continue to flow during maintained depolarization. The maximum amplitude of the Ca 2+ current (∼100 μA/cm 2 of membrane area) is usually smaller than the Na + or K + currents in axons.

The relationship between Ca 2+ channel open probability and V m is similar to that for voltage-gated Na + channels, except that the channels activate over a more depolarized voltage range. In some cell types, voltage-gated Ca 2+ channels can generate calcium action potentials (Ca 2+ APs) in a manner similar to Na + channels. A depolarizing stimulus opens Ca 2+ channels, and Ca 2+ ions flow into the cell down their electrochemical gradient. This Ca 2+ current further depolarizes the cell and opens more channels. The positive feedback of membrane depolarization on channel opening produces the Ca 2+ AP upstroke. Compared with axonal APs generated by Na + channels, pure Ca 2+ APs have slower upstrokes and longer durations because the Ca 2+ channels activate more slowly than Na + channels, and inactivation is slow and incomplete. Ca 2+ APs also have lower amplitudes than Na + APs.
Ca 2+ channel blockers are useful therapeutic agents
Various drugs reduce Ca 2+ currents by blocking voltage-gated Ca 2+ channels. These Ca 2+ antagonists include phenylalkylamines (e.g., verapamil and its methoxy derivative known as D600 or gallopamil), benzothiazepines (e.g., diltiazem), and the dihydropyridine derivatives (e.g., nifedipine, nimodipine, and nicardipine). At physiological pH, D600 and diltiazem are protonated and therefore positively charged, whereas nifedipine is uncharged. These drugs selectively block L-type Ca 2+ channels ( Box 8.1 ).
Ca 2+ channel blockers are widely used as therapeutic agents in the management of coronary artery disease, hypertension, and cardiac arrhythmias ( Box 8.3 ). Some properties of the block produced by Ca 2+ antagonists are shown in Fig. 8.3 . The data in Fig. 8.3 A illustrate the effect of repetitive depolarizations on the development of Ca 2+ channel block by the positively charged D600. Very little reduction in I Ca occurs in response to the first stimulus after D600 is added ( Fig. 8.3 A). Subsequent depolarizations, however, produce smaller and smaller Ca 2+ currents. This behavior is called use-dependent block of the Ca 2+ channels. In contrast, uncharged drugs, such as nifedipine, block Ca 2+ channels without requiring depolarization; that is, they produce a significant degree of tonic block and very little use-dependent block. Diltiazem is intermediate between D600 and nifedipine in its use dependence. Use-dependent block by charged drugs is explained by the idea that the activation gate in the channel must open before charged drug molecules can gain access to the blocking site ( Fig. 8.3 B).
The force generated by contracting cardiac or vascular smooth muscle cells depends on [Ca 2+ ] i . A major pathway for Ca 2+ entry into these cells is through voltage-gated Ca 2+ channels. Block of Ca 2+ channels with a Ca 2+ antagonist drug decreases Ca 2+ entry into the cell, and thereby decreases the force of contraction. The relaxation of vascular smooth muscle results in arterial vasodilation. This effect underlies the rationale for using Ca 2+ channel blockers to treat hypertension (high blood pressure). Chronic hypertension is the result of increased vascular resistance. Ca 2+ channel blockers reduce blood pressure by relaxing arteriolar smooth muscle and decreasing vascular resistance. Ca 2+ antagonists are also used in the treatment of angina pectoris (chest pain arising from inadequate blood supply to heart muscle). The benefits may result from coronary artery dilation (and consequently increased blood supply) or from decreased myocardial oxygen consumption that is secondary to decreased cardiac contractility.

Ca 2+ release-activated Ca 2+ (CRAC) channels are opened by depletion of endoplasmic reticulum Ca 2+ stores
Store-operated channels (SOCs) are plasma membrane Ca 2+ channels that are activated by the depletion of Ca 2+ stores in the endoplasmic reticulum (ER). The currents through these channels were initially studied by depleting Ca 2+ from the ER, and are referred to as Ca 2+ release-activated Ca 2+ (CRAC) channel currents. CRAC channels have high Ca 2+ selectivity, low single-channel conductance, and are inhibited by lanthanide cations. These properties were ultimately the criteria used to identify the protein ORAI1 as a CRAC channel. ORAI1 is a 33 kDa plasma membrane protein with four transmembrane α helices, and the functional channel may be composed of six ORAI1 subunits. ORAI1 channels are activated by the binding of stromal interaction molecule 1 (STIM1), which is a transmembrane protein located in ER membranes. STIM1 serves two functions in activating ORAI1 channels: it acts as the Ca 2+ sensor by detecting depletion of ER Ca 2+ stores, and it communicates this information to the surface membrane by binding to, and activating, ORAI1 channels. Homologues, ORAI2, ORAI3, and STIM2, have been identified. CRAC channels serve a variety of physiological functions in different cell types. For example, in lymphocytes the channels play a role in causing the rise in [Ca 2+ ] i that is required for Ca 2+ -dependent gene transcription. In skeletal muscle, STIM1 and ORAI1 are located close to one another at triads ( Chapter 15 ), where they allow fast Ca 2+ entry during store depletion. Mutant mice lacking STIM1 undergo rapid skeletal muscle fatigue because of the absence of store-operated Ca 2+ entry. CRAC channels also play a role in replenishing Ca 2+ stores in smooth muscle ( Chapter 15 ).
Many members of the transient receptor potential superfamily of channels mediate Ca 2+ entry
The first member of the transient receptor potential (TRP) superfamily of ion channels to be identified was the Drosophila TRP channel, which mediates a transient electrical response to light in photoreceptors. Six families of mammalian TRP channel proteins, containing a total of 28 TRP channels, have been identified based on sequence homology: TRP canonical (TRPC), TRP vanilloid (TRPV), TRP melastatin (TRPM), TRP polycystin (TRPP), TRP mucolipin (TRPML), and TRP ankyrin (TRPA). Three of these, TRPV1, TRPM8, and TRPA1, are involved in detection of hot and cold and in chemosensation ( Box 8.4 ). All TRP proteins contain six transmembrane domains; these proteins assemble as homotetramers or heterotetramers to form nonselective cation channels. TRP channel subtypes vary widely in their permeability to monovalent and divalent cations. Many of these channels conduct Ca 2+ and thus contribute to rises in [Ca 2+ ] i .
Members of the transient receptor potential (TRP) superfamily of channels are involved in the detection of hot and cold. Interestingly, the same channels respond to chemicals (e.g., capsaicin and menthol) that produce hot or cold sensations.
TRPV1 channels are involved in the detection of heat
The TRPV family is made up of four mammalian subfamilies: TRPV1/2, TRPV3, TRPV4, and TRPV5/6. TRPV1 channels were first identified in primary somatosensory neurons in dorsal root and trigeminal ganglia. They are ligand-gated nonselective cation channels. TRPV1 channels contribute to the sensation of heat, either in the form of increased temperature or by the presence of chemicals that produce a hot feeling. The channel is activated by elevated temperatures (≥43°C) or by capsaicin, which is the pungent essence of hot chili peppers.
TRPM8 channels are activated by cold or by cooling chemicals
There are four subfamilies of TRPM channels: TRPM1/3, TRPM2/8, TRPM4/5, and TRPM6/7. TRPM8 is expressed in very high levels in sensory neurons. TRPM8 channels function as PM Ca 2+ channels. The channel is activated by low temperature (8°C to 28°C) or by chemicals, such as menthol, that evoke a cooling sensation.
TRPA1 channels are activated by pungent chemicals
The TRPA family contains only a single member, TRPA1, which is expressed in somatosensory neurons in dorsal root and trigeminal ganglia. TRPA1 is activated by garlic and by isothiocyanate compounds, which include the pungent ingredients in wasabi, horseradish, and mustard oil.
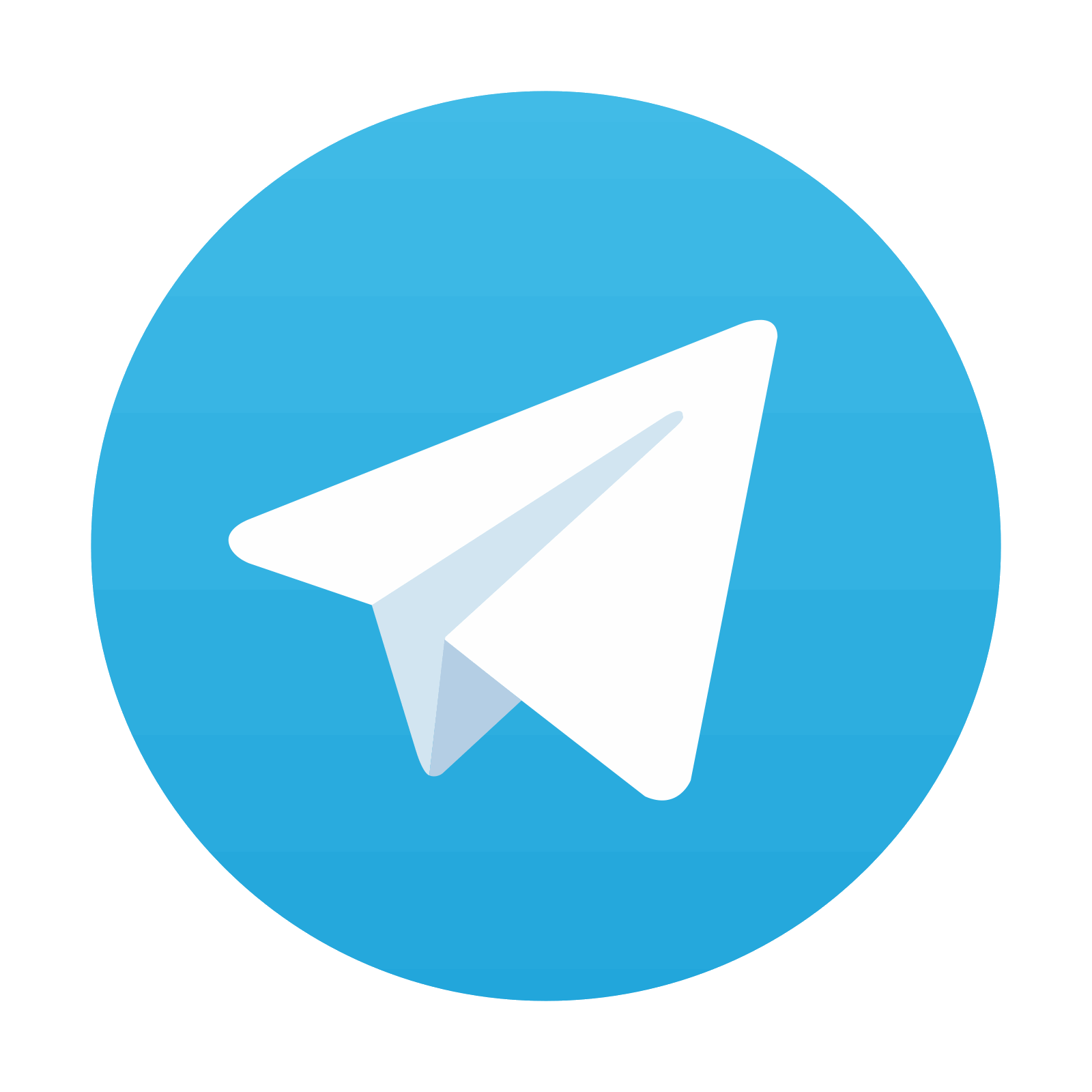
Stay updated, free articles. Join our Telegram channel
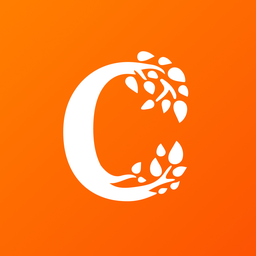
Full access? Get Clinical Tree
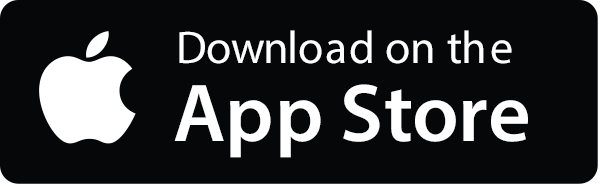
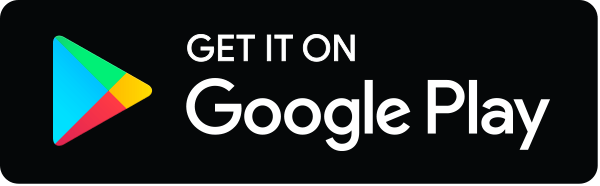
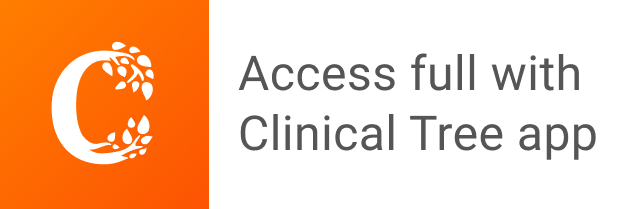