Chapter 11 Low-intensity laser therapy
BRIEF HISTORY
Lasers have found a range of applications in medicine and particularly in surgery: ophthalmic surgeons were the first to use the pulsed ruby laser successfully for the treatment of detached retina in humans. In general, most medical applications to date have relied upon the photothermal (heating) and photoablative (‘explosive’) interactions of laser with tissue; thus lasers are routinely used to cut, weld and even destroy tissue. The widespread use of lasers as alternatives to metal scalpels, as well as for tumour ablation and tattoo removal, are all based upon these tissue reactions. In parallel with such developments, early interest also focused on the potential clinical applications of the non-thermal interactions of laser light with tissue, principally based upon initial work carried out by Professor Endre Mester’s group in Budapest during the late 1960s and early 1970s. Results of this work indicated the potential of relatively low-intensity laser irradiation applied directly to tissue to modulate certain biological processes – in particular to photobiostimulate wound-healing processes (Mester et al 1985). Based on Mester’s work in animals, and subsequently in patients, the ensuing decade saw the promotion of irradiation using the He-Ne laser (a laser device based upon a mixture of helium and neon gases as the lasing medium) as the treatment of choice for a variety of conditions throughout the countries of the former Soviet Union and in Asia, particularly China. However, it was not until the introduction of small, compact, laser-emitting photodiodes that the use of this therapy, known as low-level or low-intensity laser therapy (LILT), started to gain popularity in the West. The modality has found application by physiotherapists (for human and animal use), dentists, acupuncturists, podiatrists and some physicians, for a range of conditions including the treatment of open wounds, soft-tissue injuries, arthritic conditions and pain associated with various aetiologies (Baxter et al 1991). More recently, a series of approvals by the Food and Drug Administration (FDA) has led to increasing usage in the USA.
DEFINITIONS AND NOMENCLATURE
‘Low-intensity laser therapy’ (Baxter 1994) and ‘low (reactive)-level laser therapy’ (Ohshiro & Calderhead 1988), are generic terms that define the therapeutic application of relatively low-output (typically <500mW for a single source) lasers and monochromatic superluminous diodes at dosages generally considered to be too low to effect any detectable heating of the irradiated tissues (usually <35Jcm−2). LILT is thus an athermal treatment modality. For this reason, this modality has also sometimes (inappropriately) been termed ‘soft’ or ‘cold’ laser therapy to distinguish the devices (and the resulting applications) from high-power sources of the type used in surgery and in other medical and dental applications; however, such terms are misleading and inappropriate, and are therefore best avoided.
PHYSICAL PRINCIPLES
Fuller accounts of the biophysical principles underpinning the therapeutic applications of lasers in LILT are available elsewhere (Nussbaum et al 2003a).
LIGHT EMISSION, ABSORPTION AND THE PRODUCTION OF LASER RADIATION
The basis of production of stimulated emission is illustrated in Figure 11.1. In non-laser sources, light is typically produced by spontaneous emission of radiation (Fig. 11.1A). In such circumstances and devices, the atoms and molecules comprising the central emitter (e.g. the element/filament in a typical household light bulb) are stimulated with (electrical) energy so that the electrons shift to higher energy orbits. Once in such orbits, the electrons are inherently unstable and fall spontaneously within a short period of time to lower energy levels. In so doing they release their extra energy as photons of light. The properties of the emitted photons are determined by the difference in energy levels (or valence bands) through which an excited electron ‘dropped’, as the difference in energy will be exactly the same as the quantal energy of the photon produced. As, for a given photon of light, the quantal energy (specified in electron-volts) is inversely related to the wavelength (in nm), the wavelength is effectively determined by the difference in valence bands; and in turn, molecules produce typical ranges of wavelengths or emission spectra when appropriately stimulated.
ABSORPTION OF RADIATION
Absorption occurs when a photon of light interacts with an atom or molecule in which the difference in energy of the valence bands exactly equals the energy carried by the photon (Fig. 11.1B). This has two consequences: for a photon of a given quantal energy (and thus wavelength) only certain molecules will be capable of absorbing the light radiation; conversely, for a given molecule, only certain quantal energies (and thus wavelengths) can be absorbed (this is termed the absorption spectrum for the molecule). Thus absorption is said to be wavelength specific. This is an important concept in LILT applications, as this wavelength specificity of absorption effectively determines which types of tissue will preferentially absorb incident radiation, and (in turn) the depth of penetration of a particular treatment unit.
STIMULATED EMISSION OF RADIATION
Stimulated emission is a unique event that occurs when an incident photon interacts with an atom that is already excited (i.e. where the electron(s) are already in a higher energy orbit); additionally, the quantal energy of the incident photon must exactly equal the difference in energy levels between the electron’s excited and resting states (Fig. 11.1C). Under these exceptional circumstances, the electron, in returning to its original orbit, gives off its excess energy as a photon of light with exactly the same properties as the incident photon, and completely in phase. In laser devices, the unique circumstances that give rise to stimulated emission of radiation are produced through the selection of an appropriate material or substance (the lasing medium), which, when electrically stimulated, will produce large numbers of identical photons through the rapid excitation of the medium. In order to produce such stimulated emission of radiation, laser treatment devices rely upon three essential components: the lasing medium, the resonating cavity and the power source.
Lasing medium
A lasing medium is capable of being ‘pumped’ with energy to produce stimulated emission; for therapeutic systems, the energy source is invariably electrical and the energy is delivered to the medium typically from the mains or (less commonly) from a battery (see below). The two media most commonly used in LILT applications are the gaseous mixture of helium and neon (He-Ne) operating at a wavelength of 632.8nm (i.e. red light) and gallium arsenide (Ga-As), or gallium–aluminium–arsenide (GaAIAs) semiconductors, which typically produce radiation at 630–950nm (i.e. visible red to the near infrared). Although He-Ne laser systems were the first to be used for LILT applications (i.e. they represented the ‘first generation’ of such systems), and a significant percentage of the papers published within this area are based upon such devices, these find few applications in routine physiotherapeutic practice. This is due to the relative expense of such units, and the comparatively low power output associated with He-Ne systems. In addition, the relatively greater collimation (see below) of these units when applied without fibreoptic applicators poses a significant hazard to the unprotected eye compared with the average semiconductor-diode-based treatment unit.
Resonating cavity
A resonating cavity or chamber consists of a structure to contain the lasing medium and incorporates a pair of parallel reflecting surfaces or mirrors. Within this chamber, photons of light produced by the medium are reflected back and forth between the ‘mirrors’ to ultimately produce an intense photon resonance. As one of the reflecting surfaces (also termed the output coupler) is not a ‘pure’ mirror and so does not reflect 100% of the light striking its surface, some of the radiation is allowed to pass through as the output of the device. The resonating cavity for diode-based units is essentially the lasing medium itself (i.e. the semiconductor diode), the ends of which are carefully polished to form reflecting surfaces. This has important implications for the routine use of these units in clinical practice, as the treatment ‘head’ or ‘probe’ is usually not much larger than the size of a pen; this represents another reason why diode-based units (which may be regarded as ‘second-generation’ laser therapy systems), are so popular with clinicians (Fig. 11.2). Furthermore, most manufacturers now offer multisource ‘cluster’ arrays, incorporating a number of diodes (up to 180) to allow simultaneous treatment of larger lesions and (in some cases) to allow several wavelengths of radiation to be used simultaneously (Fig. 11.2B). Such multisource cluster units may be regarded as the ‘third generation’ of development for therapeutic lasers. More recently, several manufacturers have introduced ‘fourth-generation’ flexible multisource arrays to allow more efficient delivery of light to tissue surfaces with ‘hands-free’ application.
CHARACTERISTICS OF LASER RADIATION
LASER-TISSUE INTERACTION
CONCEPTUAL BASIS OF LASER PHOTOBIOMODULATION: THE ARNDT-SCHULTZ LAW
The photobiological effects of laser or monochromatic light upon tissue are many and complex, and to a large degree still poorly understood, particularly in terms of the variable stimulative/inhibitory reactions that can be effected by such irradiation. In providing a theoretical basis for the observed biological and clinical effects of this modality, the Arndt-Schultz law has been proposed as a suitable model; the main tenets of this law are illustrated in Figure 11.3. It should be stressed, however, that although this model can account for such phenomena as the ‘inverse’ dosage dependency reported in some papers (e.g. Lowe et al 1994), it essentially applies to radiant exposure (or energy density – see below); the putative relevance of manipulation of other irradiation parameters such as pulse repetition rate or power output remains, at least for the time being, a matter of debate (Nussbaum et al 2003b).
BIOLOGICAL AND PHYSIOLOGICAL EFFECTS
Although the following provides an overview of the findings to date in these areas, a full and comprehensive review of the literature on the biological and physiological effects of low-intensity laser radiation is beyond the scope of this chapter; for further detail the reader is directed to the reviews of Basford (1995), Baxter (1994), Karu (1998), Schindl et al (2000), Shields and O’Kane (1994) and Tuner and Hode (1999).
CELLULAR RESEARCH
A large number of studies have examined the biological basis of low-intensity laser irradiation using a variety of bacteria, as well as cell lines and explanted cells, especially fibroblasts and macrophages (e.g. Pogrel et al 1997). In these studies, a number of possible indicators have been used to assess the photobiomodulatory effects of laser irradiation, including cell proliferation (Bolton et al 1995, Hawkins & Abrahamse 2006, Stein et al 2005), collagen production (Medrado et al 2003) and gene expression (Byrnes et al 2005). However, it should be stressed that although results from such studies are generally positive, findings in some areas are equivocal (Basford 1995, Posten et al 2005).
Cellular studies are important in two respects. In the first instance they provide a scientific basis for the clinical application of low-intensity lasers for the management of wounds, through demonstration of the photobiological mechanisms underlying such treatments (Karu 1998, Posten et al 2005). Second, by using such well-controlled laboratory research techniques, systematic investigations by some groups have demonstrated the importance of laser irradiation parameters such as wavelength, dosage and pulse repetition rate to the observed effects (O’Kane et al 1994, Rajaratnam et al 1994).
This notwithstanding, the precise relevance of the reported observations to clinical treatments is not always entirely clear. For example, where photobiostimulatory effects might be reported at a radiant exposure of 1.5Jcm−2 in a laboratory study involving the direct irradiation of artificially maintained murine macrophage-like cell lines, what direct relevance has this for dosage selection in the laser treatment of a venous ulcer in a 67-year-old patient? Given such problems, e.g. the differences between the cell lines and the highly complex microenvironment of the clinical wound, a number of groups have employed animal studies and experimental studies on healthy human volunteers to further assess the biological and physiological effects of this modality in the laboratory.
ANIMAL STUDIES
To date, animal studies have concentrated on two main areas of research: the photobiostimulatory effects of laser irradiation on wound healing in experimentally induced lesions, and the neurophysiological and antinociceptive (‘pain-blocking’) effects of such irradiation. For the former studies, small, loose-skinned animals such as rats and mice have been traditionally, and most commonly, used (e.g. Mester et al 1985, Rabelo et al 2006). A range of experimental wounds have been employed in these species, including bone defects (Khadra et al 2004), burns (Bayat et al 2006), tendon and ligamentous injuries (Bayat et al 2005) and open skin wounds based upon models of compromised wound healing (Mester et al 1985, Rabelo et al 2006, Walker et al 2000). Although such studies have typically reported positive effects of laser irradiation (in terms of increased rates of healing, wound closure, enhanced granulation tissue formation, etc; see Woodruff et al 2004), the quality of much of the literature to date has been criticized (Lucas et al 2002) and the experimental lesions in these animals have long been considered to represent a poor model for wounds in humans, because of the species differences in tegument compared with humans (King 1990).
Perhaps the most interesting aspect of this type of animal work has been reports of the potential of laser irradiation to accelerate regeneration of damaged nerves, together with associated electrophysiological and functional recovery, after various types of experimental lesions (Anders et al 2004). If such effects are also possible in humans, the implications for future applications of this modality would be enormous (Gigo-Benato et al 2005).
The effects of laser irradiation have been studied in various animal models of pain, to investigate its mechanism of action, and dependence upon irradiation parameters (Ferreira et al 2005, Ponnudurai et al 1987, Wedlock & Shephard 1996, Wedlock et al 1996). These studies have generally demonstrated a significant hypoalgesic effect of laser irradiation, which seems to be dependent upon the irradiation parameters used (pulse repetition rate: Ponnudurai et al 1987; dosage: Wedlock et al 1996). Furthermore, the observed hypoalgesia has been found to be naloxone reversible in some studies (Wedlock & Shephard 1996), but not in others (Ponnudurai et al 1987), suggesting that the pain relief obtained with laser irradiation may be underpinned by a variety of different mechanisms, and in some circumstances may be opiate mediated.
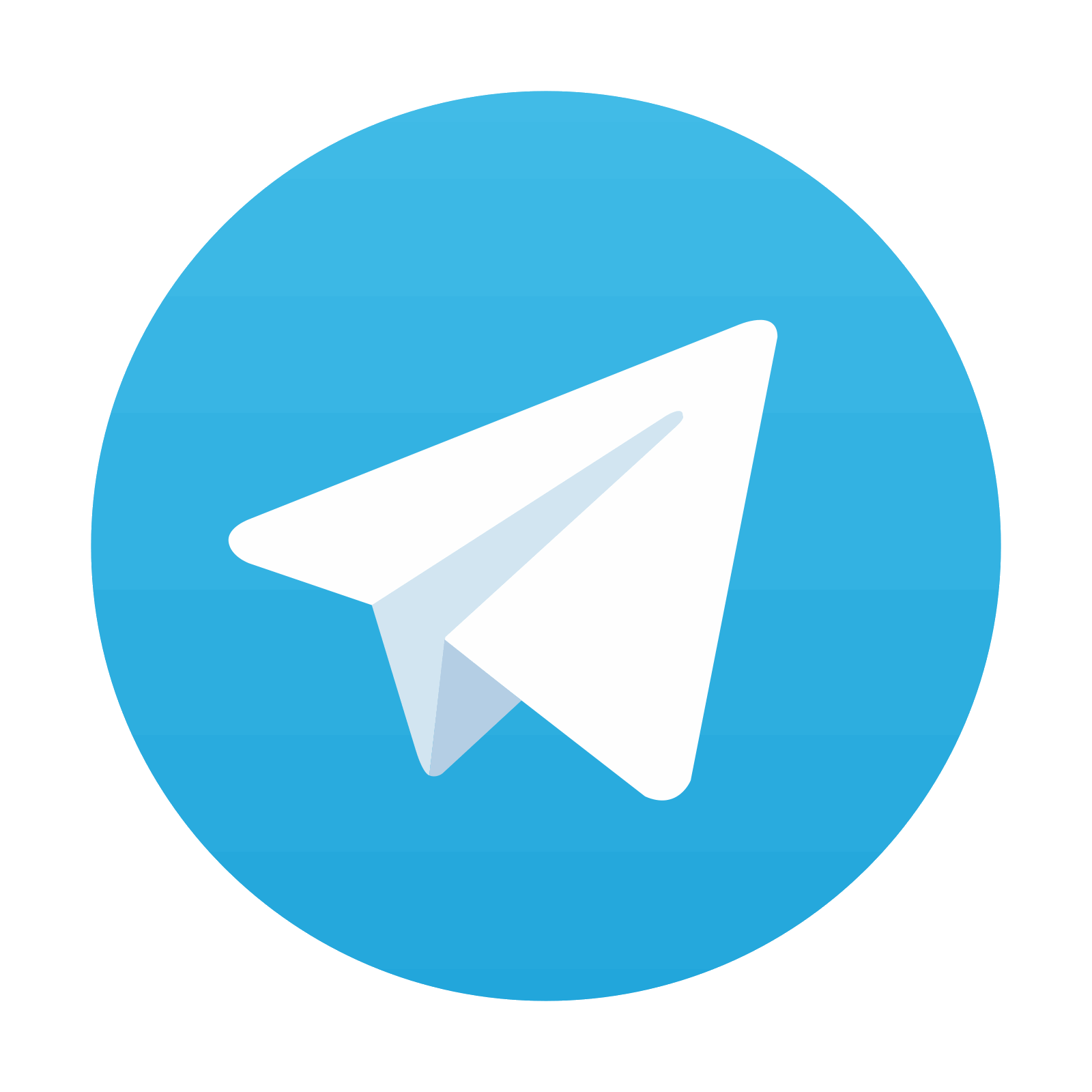
Stay updated, free articles. Join our Telegram channel
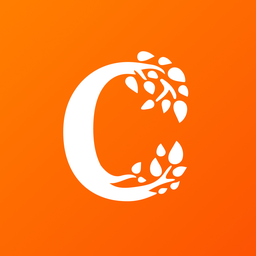
Full access? Get Clinical Tree
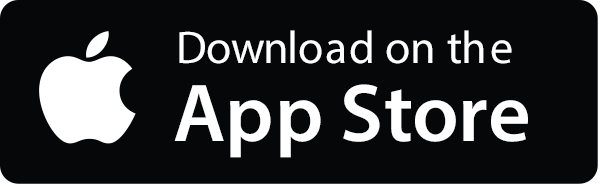
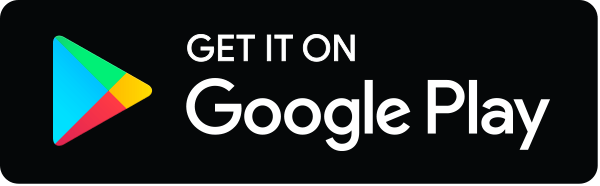