Study population
No of subjects
Type of XR Li
Reference
Observed pharmacokinetics parameters
Normal control subjects
6
Lithium carbonate LP 400 mg (after 12 h)
Hunter (1988)
C max = 1.49 ± 0.31 mmol/L
T max = 2.1 ± 0.5 h
t 1/2 = 27.6 ± 9.1 h
V = 50.9 L
CL/F = 1.25 ± 0.4 L/h
ASC (0–24) = 22.8 ± 4.9 mmol/L/h
Normal control subjects
11
Lithium carbonate LP 450 mg (after 12 h)
Kristoff et al. (1986)
15 % increase in concentration with ibuprofen 400 mg co-prescription
Normal control subjects
16
Lithium acetate 536 mg (after 12 h)
Turck et al. (2000)
21 % increase in concentration and AUC with meloxicam (20 mg) co-prescription
Lithium PK parameters (XR lithium monotherapy group):
C pre, ss = 0.54 mmol/L
C max, ss = 0.97 mmol/L
ASC ss = 7.75 mmol/L/h
T max, ss = 1.5 h,
C av = 0.66 mmol/L
C min, ss = 0.35 mmol/L
CL/F = 30.2 mL/min,
PTF = 96 %
Ae = 14.0 mmol (100 % of dose)
CLr = 30.2
Bipolar patients (including 31 subjects with lithium toxicity)
50
Lithium carbonate LP 400 mg or 200 mg (after 12 h)
ElDesoky et al. (2008)
CL = 0.51 L/h with 12.7 % BSV
V1 (fixed) = 15.2 L
Q (fixed) = 7.44 L/h
V2 (fixed) = 6.7 L
Bipolar patients
42
Lithium LP citrate (after 24 h)
Hoegberg et al. (2012)
Comparison of lithium carbonate IR and lithium citrate
Fr = 0.33 vs 0.33
V1 (L⁄kg) = 0.74 vs 0.74
ka (/h) = 2.79 vs 0.75
f = 1.00 vs 0.85
DS (mM) = 0.0036 vs 0.006
Overall, the literature does not contain any comprehensive PK studies of XR lithium (once daily). The plasma lithium therapeutic range used in clinical practice and measured at 12 h is based on approximate estimations of lithium plasma targets from old studies of IR lithium formulations (residual concentration).
The PK-PD relationship for XR lithium is another understudied area. Most of the published data have studied the PK-PD relationships for the IR formulation of lithium, and the results are sometimes contradictory (Higuchi et al. 1988; Terao et al. 1999; Wright and Crismon 2000; Chiu et al. 2007; Abou-Auda et al. 2008; Hoegberg et al. 2012; Huang et al. 2008; Sproule 2002). Indeed, the relationship between the residual concentration (Cmin) and the therapeutic response is poorly characterized. The proportion of responders (relapse prevention) increases proportionally with the increases in the Cmin to approximately 0.8–1.0 mmol/L (Sproule 2002). However, these concentrations are close to toxic concentrations (≥1.5 mmol/L) (Simard et al. 1989). A significant proportion of patients exhibit documented response to lithium despite low plasma concentrations (approximately 0.4–0.7 mmol/L) (Coppen et al. 1973). Furthermore, toxicity has been observed in some patients with Cmin within the recommended therapeutic range (Sproule 2002). Several parameters influencing the Cmin are likely to influence the therapeutic response (Amdisen 1977; Greil et al. 1985; Swartz 1987). Minimal Cmin fluctuations can exert significant effects on efficacy and safety (Amdisen 1977; Greil et al. 1985; Swartz 1987). In addition, discrepancies between the plasma and erythrocyte concentrations of lithium may exist in some patients and be a source of toxicity or ineffective treatment (Frazer et al. 1973). Altogether, these data clearly suggest that the study of PK-PD relationships is hampered by the complex architecture of factors influencing lithium’s PK and PD, heterogeneity of bipolar disorders with regard to lithium response, and narrow therapeutic range. These results further suggest that the estimations of the PK parameters and the PK-PD relationships of XR lithium based on IR lithium results are highly speculative. Specific PK studies of XR lithium using modern population PK modelling must be performed to identify not only the PK model parameters but also their variability.
9.5 Brain Lithium Pharmacokinetics
Animal studies provide abundant direct and indirect evidence to support regional variations in the brain distribution of lithium. Twenty-four hours after a single subcutaneous lithium injection, heterogeneous lithium distribution was observed among rat brain regions, with the hypothalamus, corpus striatum, and midbrain being the regions with the highest lithium accumulation (Rios and Guzman-Mendez 1990). After the administration of 3 mEq/kg lithium chloride, the hypothalamus exhibited the highest concentration between 0.5 and 8 h, and after 8 h, the highest concentration was observed in the caudate nucleus, followed by the cortex and rest of the diencephalon (Mukherjee et al. 1976). Heterogeneous lithium distribution in rat brain regions at therapeutic dosages after prolonged treatment has also been reported (Ramaprasad 2004). Significant differences were found in vivo among lithium concentration ratios for the various brain regions, using either lithium-7 (7Li) MR spectroscopic imaging technology or in vitro methods (Komoroski et al. 1997).
Not only is lithium’s brain distribution heterogeneous, but its activity at a molecular level also exhibits high variability. Lithium appears to be most active in a region stretching from the anterior cingulate cortex and striatum to the caudal midbrain, with the greatest activity in the preoptic area and hypothalamic regions (Ramaprasad et al. 2005). Only some activity was observed in prefrontal cortex, and the lowest molecular activity was observed in the cerebellum and metencephalic brainstem. Accordingly, in rats chronically fed with a diet containing 0.2 % lithium carbonate, myo-inositol monophosphatase-1 activity increased substantially in various brain regions analysed, even doubling in some regions, in the following order: hippocampus > cerebellum > striatum > cerebral cortex > brainstem (Parthasarathy et al. 2003). In addition, the effect of 1, 2 and 4 weeks of lithium treatment (1 mmol/kg/day, intraperitoneal) on the myo-inositol concentrations across several rat brain regions, including the prefrontal, temporal and occipital cortical areas, as well as the hippocampus, was analysed using MRS at 18.8 T (McGrath et al. 2006). However, while other studies suggest brain region-specific alterations in myo-inositol concentrations among bipolar patients, this study found that lithium-induced reduction of myo-inositol is more global.
Excellent opportunities have emerged using MRS at high magnetic fields to image brain lithium-7 with unprecedented sensitivity (Lee et al. 2012; Renshaw et al. 1985). Lithium’s distribution in the brain, the mechanisms influencing lithium brain distribution, and lithium’s mechanism of molecular action in these different regions are important questions that need to be addressed, and answers to these questions may help to identify sensitive and specific biomarkers of lithium response.
The brain pharmacokinetics of lithium does not follow its plasma pharmacokinetics (time to reach maximal concentration and elimination half-life), suggesting active mechanisms underlying the distribution of lithium through the brain barriers; to date, the role of the blood-brain barrier (BBB) and the blood-cerebrospinal fluid barrier (BCSFB) in brain lithium PK-PD relationships has never been studied. Moreover, these transport mechanisms through the brain barriers, due to their region-specific expression and/or activity, may be responsible for the heterogeneity in lithium’s brain distribution. Interestingly, the Na+-dependent Cl−/HCO3 − exchanger (NCBE) encoded by the SLC4A10 gene is highly expressed and is of major importance in the physiology of choroid plexuses for the production of cerebrospinal fluid (CSF) (Damkier and Praetorius 2012). This Na+ exchanger likely transports lithium, and, recently, the SLC4A10 gene has been suggested in a GWAS study as a candidate gene for lithium response in bipolar disorder (Rybakowski 2013).
9.6 Molecular Signature of Lithium Response
Lithium’s mechanism of action is rather complex because it exerts inhibitory and facilitatory actions on several cellular signalling pathways. In the brain, lithium acts on pre- and postsynaptic neurons and has been demonstrated to inhibit excitatory transmission and to promote inhibitory transmission. Its modulatory effects on dopamine, glutamate and GABA neurotransmissions have been investigated in animal and clinical studies (Ahluwalia et al. 1981; Vargas et al. 1998; Ferrie et al. 2005, 2006). Lithium inhibition of the solute carrier family 5 member 3 (SLC5A3)-mediated entry of inositol into the cells and direct inhibition of inositol monophosphatase (IMPase) and inositol polyphosphate 1-phosphatase (IPPase) (Gould and Manji 2002; Willmroth et al. 2007) contribute to a decrease in intracellular inositol, therefore inhibiting protein kinase C (PKC)-associated signalling and reducing excitatory neurotransmission (Machado-Vieira et al. 2009; Lenox and Wang 2003; Zarate and Manji 2009; Lenox et al. 1992). Lithium also directly inhibits GSK3β, which in turn activates the Akt pathway (Klein and Melton 1996; Tajes et al. 2009). Another pathway affected by lithium is the cAMP pathway, resulting in an increase in the basal levels of cAMP and adenylate cyclase (AC) (Mann et al. 2009; Marmol 2008; Quiroz et al. 2010). All of these targets and effectors contribute to the neuroprotective, neuroproliferative and mood stabilizing actions of lithium in the brain and complicate the understanding of the lithium prophylactic response.
To understand the variability in responses to lithium, a complementary approach to genetic studies is to study lithium’s molecular effects. Lithium has several cellular targets and modulates expression levels at the transcriptomic, proteomic and also miRNA levels in several in vitro and in vivo models.
9.6.1 Animal Models
Available studies on lithium’s molecular effects in animal models are summarized in Table 9.2. The short-term effects of lithium on the proteome have been analysed after acute lithium intraperitoneal injection, and an increase was observed in the levels of fourteen proteins in the rat hypothalamus (Lee et al. 2009). Acute lithium has also been demonstrated to increase the mRNA levels of the immediate early gene early growth response 1 (Egr1) and the circadian gene period circadian clock 2 (Per2) in mice frontal cortices (Kim et al. 2013). In mice, chronic lithium was found to modulate 121 mRNAs in the brain, including Per2 (McQuillin et al. 2007); these results are in agreement with the observed impact of lithium on circadian rhythms in rodents (Abe et al. 2000; Subramanian et al. 1998; Williams and Jope 1995).
Table 9.2
Available studies on lithium’s molecular effects in animal models
Species | Brain region | Dose | Duration of treatment | Route of administration | Observed modulations | Reference |
---|---|---|---|---|---|---|
Rat | Cerebral cortex | 0.24 % | 4 weeks | Food | ↑ AC mRNA ↓ Giα mRNA | Colin et al. (1991) |
Rat | Hippocampus Temporal cortex Frontal cortex | 0.2 % | 14 days 28 days | Food | ↑ BDNF peptide | Fukumoto et al. (2001) |
Rat | Hippocampus Cerebral cortex | 0.2 % | 4 weeks | Food | ↑ IMPase 1 protein | Parthasarathy et al. (2003) |
Rat | Choroid plexus | 75 mg/kg | 28 days | Osmotic pump | ↓ Ttr mRNA | Pulford et al. (2006) |
Rat | Prefrontal cortex | 22 mg/kg | 28 days | i.p. | ↓ Gpx4, Goα, tubulin β proteins ↑ Syn1 protein | Corena-Mcleod Mdel et al. (2008) |
Rat | Hypothalamus | 0.15 M | 1 h and 6 h | i.p. | ↑ 14 proteins at 1 h ↑ 32 proteins at 6 h | Lee et al. (2009) |
Rat | Hippocampus | 2.4 g/kg | 4 weeks | Food | ↓ Let-7b, let-7c, miR-128a, miR-24a, miR-30c, miR-34a, miR-221 miRNAs ↑ miR-144 miRNAs | Zhou et al. (2009) |
Rat | Cortex | 120 mg/kg | 10 days | i.p. | ↑ Adra2A mRNA | Cuffi et al. (2010) |
Rat | Frontal cortex | 50 mg/kg | 21 days | i.p. | ↓ Pde4ax and Pde4b1 proteins | Fatemi et al. (2010) |
Rat | Hippocampus | 0.1 % | 4 weeks | Food | ↑ 104genes (mRNA) ↓ 96 genes (mRNA) | Lee et al. (2015) |
Mice | Brain | 0.4 % | 2 weeks | Food | 121 genes (mRNA) | McQuillin et al. (2007) |
Mice | Frontal cortex | 150 mg/kg | 2 h | i.p. | ↑ Per2 and Egr1 mRNA | Kim et al. (2013) |
Mice | Microglia | 600 mg/L | 2 weeks | Water | ↑ 104genes (mRNA) ↓ 126 genes (mRNA) | Yu et al. (2015) |
Because lithium is a long-term treatment, most of the reported studies have focused on lithium-induced modulations after chronic treatment. In rats, chronic lithium treatment has been demonstrated to modulate the mRNA levels of several genes in the brain. In line with lithium’s effects on the cAMP pathway, the upregulation of AC and downregulation of Giα were observed in the rat cerebral cortex after chronic treatment with lithium (Colin et al. 1991). In the prefrontal cortex, a comparative study of the effects of lithium, valproate and paliperidone found that only the levels of synapsin I (Syn1) were increased by the three drugs; in contrast, lithium decreased specifically Goα, tubulin-β and glutathione peroxidase 4 (Gpx4) protein levels (Corena-McLeod Mdel et al. 2008). Another comparative study found that the expression of two isoforms of phosphodiesterase (PE) (PE4B and PE4E) decreased in the rat frontal cortex after lithium, clozapine, haloperidol and valproate chronic treatment, while lithium alone reduced the mRNA levels of PDE8B, suggesting a potential common therapeutic pathway for these psychotropic medications (Fatemi et al. 2010). Comparative transcriptomic effects of lithium and valproate in the rat hippocampus have also been recently studied (Lee et al. 2015). Lithium impact on gene expression was found more limited than that of valproate, and only few genes were found to be regulated by both drugs. In agreement with the observed increase in BDNF peptide and anti-apoptotic genes levels in the peripheral blood of lithium responders (Tramontina et al. 2009; Lowthert et al. 2012; Beech et al. 2014), an increase in the peptide levels of BDNF in the rat hippocampus and temporal cortex was observed after chronic lithium treatment (Fukumoto et al. 2001). Chronic lithium can also regulate the protein level and activity of IMPase 1, as observed in the rat hippocampus and cerebral cortex (Parthasarathy et al. 2003). Pulford and collaborators reported the downregulation of transthyretin (Ttr) mRNA in the choroid plexus (Pulford et al. 2006). Lithium treatment has been demonstrated to upregulate the transcription of the adrenoceptor alpha 2A (AdrA2a) gene in the rat cortex (Cuffi et al. 2010). In mice microglia, lithium has been shown to modulate the expression level of several genes, among these the complement component 3 (C3) was found to be the only gene significantly regulated in the mice microglia and human monocyte-derived dendritic cells (Yu et al. 2015).
A possible mechanism for the regulation of mRNA, and therefore protein, levels is the modulation of miRNA levels (Guarnieri and DiLeone 2008). A study of the impact of chronic lithium on miRNA levels in the rat hippocampus found a decrease in eight miRNAs and an increase in miR-144 (Zhou et al. 2009). This result suggests that lithium could exert some of its molecular effects through this epigenetic mechanism, which opens a new field of research. An effect of lithium on histone modifications in the leptin receptor gene Lepr has been recently found in rat hippocampus emphasizing the need to study the epigenetic impacts of this drug (Lee et al. 2015).
Animal models possess the added benefit of allowing the study of lithium effects in targeted areas of the brain. These studies have confirmed some of the observed modulations of protein and mRNA levels in patients and also initiated new research directions. However, the dissimilar routes of administration used (infusion by osmotic pump, intraperitoneal injection, lithium in food pellets, etc.) and the differences in the lithium doses and treatment duration (from 22 to 120 mg/day during 1 h to 28 days) make it difficult to compare the results.
9.6.2 Cellular Models
Several animal and human cellular models have been used to further analyse the molecular response to lithium. Available studies are summarized in Table 9.3. In line with the in vivo results, lithium-induced increases in the Bcl2 and Bdnf mRNA levels were observed in rat cultured astrocytes, cortical or hippocampal neurons (Yasuda et al. 2009; Keshavarz et al. 2013; Dwivedi and Zhang 2014). The decrease in one of the myristoylated alanine-rich PKC substrates (MARCKS) observed in the rat brain was also observed in immortalized hippocampal cells from mice (Wang et al. 2001). In line with the effects of lithium on the circadian rhythms of rodents, the molecular effects of lithium on several circadian genes have been analysed in the fibroblast cell line NIH-3T3, and significant modulations of seven genes were observed (Osland et al. 2011).
Table 9.3
Available studies on lithium’s molecular effects in cellular models
Species | Cellular model | Dose | Duration of treatment | Observed modulations | Reference |
---|---|---|---|---|---|
Rat | Cortical neurons | 1 mM | 48 h | ↑ Promoter IV Bdnf mRNA | Yasuda et al. (2009) |
Rat | Astrocytes | 1 mM | 7 days | ↑ Bcl2 mRNA | Keshavarz et al. (2013) |
Rat | Hippocampal neurons | 1 mM | 48 h | ↑ Promoter IV Bdnf, Bcl2, Bcl2l1 mRNA ↓Bad, Casp3 mRNA | Dwivedi and Zhang (2015) |
Mice | HN33 | 5 mM | 7 days | ↓ Marcks mRNA | Wang et al. (2001) |
Mice | NIH 3T3 | 20 mM | 3 days | ↑ Per2 and Cry1 mRNA ↓ Per3, Cry2, Bmal1, E4BP4 and Rev-Erb-α mRNAs | Osland et al. (2011) |
Human | LCL BP vs control | 1 mM | 7 days | ↓ ADRA1B, CHRNA1, PDE4D, SPR, and RAB7 mRNA | Sun et al. (2004) |
Human | LCLs LiR vs unaffected relatives vs controls | 1 mM | 7 days | ↓ BDNF peptide | Tseng et al. (2008) |
Human | SK-N-AS | 1.5 mM | 33 days | ↑ 347 genes ↓ 324 genes | Seelan et al. (2008) |
Human | LCL BP vs discordant siblings | 1 mM | 4, 7 and 21 days | ↑ miR-221, miR-152, miR-155, miR-24a miRNAs | Chen et al. (2009) |
Human | LCL Control ± lithium or val | 0.75 mM | 7 days | 44 genes modulated by lithium and 416 by val (18 in common) | Sugawara et al. (2010) |
Human | LCL LiR vs LiNR | 1 mM | 7 days | Modulation of SYN2a and SYN2b mRNAs | Cruceanu et al. (2012) |
Human | LCL LiR vs LiNR | 1 mM | 7 days | No significant effects of Li IGF1 differentially expressed between R and NR | Squassina et al. (2013) |
Human | iPSCs BP and control | 1 mM | 24 h | ↑ EMX2 protein | Chen et al. (2014) |
Human | LCL BP vs controls | 1 mM | 3 weeks | ↓ DBP mRNA | Kittel-Schneider et al. (2015) |
Human | LCL LiR vs LiNR | 1 mM | 7 days | ↓ 10 members of Let-7miRNAs family in R | Hunsberger et al. (2015) |
Human cellular models have also been used, and despite some inherent limitations resulting from cellular changes induced by transformation with the Epstein-Barr virus, lymphoblastoid cell lines (LCLs) have been extensively used for the identification of genes associated with bipolar disorder. Gene expression analyses in this model have identified differential gene expression in bipolar patients compared with controls or monozygotic asymptomatic twins (Matigian et al. 2007; Kato et al. 2011). In line with the impact of lithium on circadian genes observed in in vivo and cellular animal models, a lithium-induced decrease of the expression level of DBP has been shown in LCL of bipolar patients and healthy controls (Kittel-Schneider et al. 2015). The genes regulated by lithium in the LCLs of bipolar patients were found to include alpha1B-adreno-ceptor (ADRA1B), acetylcholine receptor protein alpha chain precursor (CHRNA1), phosphodiesterase 4D, cAMP-specific (PDE4D), substance P-receptor (SPR), and the ras-related protein RAB7 (Sun et al. 2004). A decrease in the BDNF peptide levels was found in LCLs from lithium-responsive bipolar patients compared with their unaffected relatives (Tseng et al. 2008).
As suggested from animal studies, lithium may exert some of its effects through miRNA modulation. miR-134 plasma levels are reported to be decreased in bipolar patients compared with controls and increased upon the introduction of medication and improvement of mood states (Rong et al. 2011), suggesting that miRNAs could be used as circulating biomarkers of lithium response variability. LCLs have been successfully used as a model to study differential miRNA modulation induced by lithium; four miRNAs (miR-221, miR-24a, miR-152 and miR-155) were found to be differentially regulated in LCLs from affected patients compared with those from their siblings (Chen et al. 2009). Using the same model, the effects of lithium in control subjects have been compared to those of another mood stabilizer valproate. This study identified 44 genes regulated by lithium and 416 by valproate and only 18 genes with mRNA levels regulated by both molecules (Sugawara et al. 2010). The gene most significantly downregulated by lithium was BCL2-associated X protein (BAX, -1, eightfold), and the most significantly upregulated gene was platelet-activating factor acetylhydrolase 1b catalytic subunit 2 (PAFAH1B2, twofold).
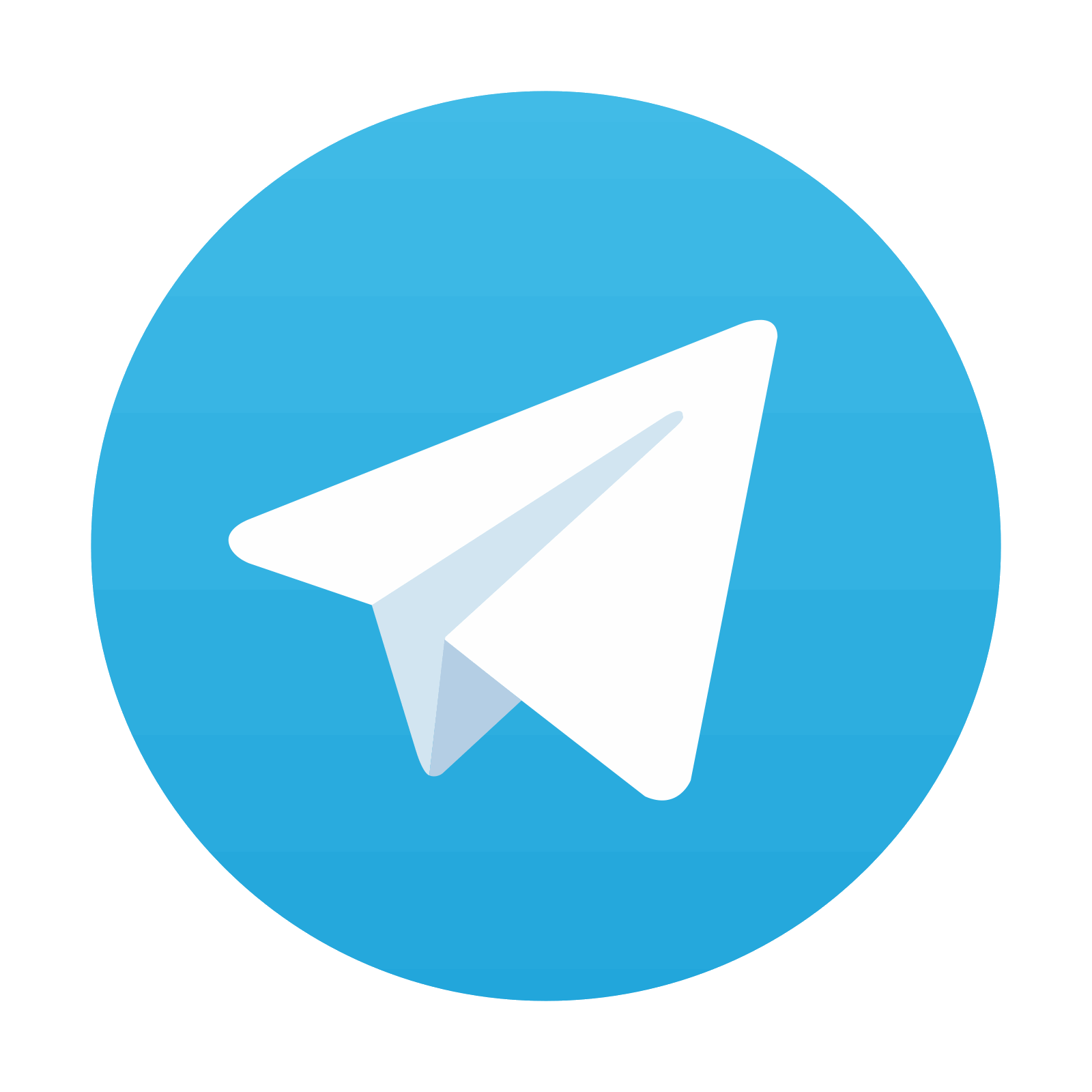
Stay updated, free articles. Join our Telegram channel
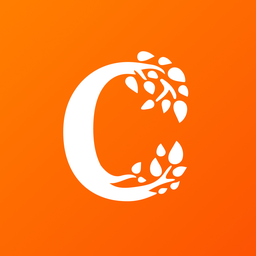
Full access? Get Clinical Tree
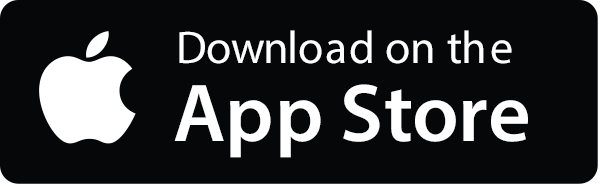
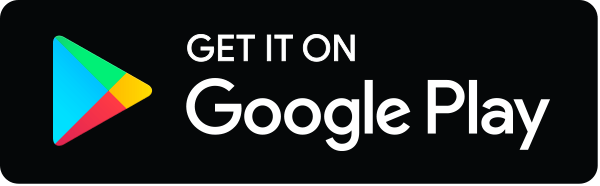