37715 Magnetoencephalography Both electroencephalography (EEG) and magnetoencephalography (MEG) obey laws of biophysics that determine the nature of the signals. MEG is a relatively new technique for visualizing brain activity. Mapping the fields of electro-magnetic brain signals reveals complementary dipolar patterns of EEG and MEG that are suited for source analysis. MEG and EEG source modeling can directly estimate the location, orientation, and strength of current dipoles. Since MEG spike fields are bipolar with both an efflux and influx maxima, MEG trace displays typically show phase reversals, both toward and away. EEG fields are also dipolar and they can be interpolated from multiple electrodes as MEG does from multiple sensors. MEG can provide a more accurate localization of certain cortical sources and that accuracy is particularly important in epilepsy surgery candidates. If there is a predominant tangential component, MEG can be very helpful in providing the correct localization. brain activity, dipolar patterns, electroencephalography, epilepsy surgery, magnetoencephalography, source modeling, spike fields, tangential component Electroencephalography, Epilepsy, Magnetoencephalography Populations of cortical neurons firing synchronously produce measurable electrical and magnetic signals in both health and disease. This chapter briefly discusses the generation of these signals, the electrical and magnetic fields that are created as a consequence, the way we measure these fields, and how we analyze them to determine their origin in the brain. The latter is of course particularly important for patients with drug-resistant epilepsy who may benefit from surgery if the focus of their seizures can be localized. Both EEG and magnetoencephalography (MEG) obey laws of biophysics that determine the nature of the signals. These result in strengths that we can exploit and weaknesses that we must understand in order to optimize information that can be used clinically. 378EEG AND MEG SIGNAL GENERATION The excitatory and inhibitory postsynaptic potentials that constitute much of neuronal activity produce an ionic sink at one end of a neuron and source at the other end with a resultant current flow through the neuron (Figure 15.1). This separation of charge is similar to the theoretical electrical dipole, with one end negative the other positive. FIGURE 15.1. Schematic of cortical pyramidal cells undergoing (A) superficial laminar depolarization with an active current sink, current flow down to the cell body, and a passive current source in deep laminae. This results in superficial extracellular negativity and deep extracellular positivity. In (B), there is a deep laminar active sink and a superficial passive source resulting in superficial extracellular positivity and deep extracellular negativity. Source: Reproduced with permission from Ebersole JS, Husain AM, Nordli DR. Current Practice of Clinical Electroencephalography. Wolters Kluwer Health; 2014. 379Similarly, the current flow through the neuron, which acts like a conductor, produces a magnetic field around it by the right-hand rule (i.e., the magnetic field encircles the neuron like the fingers of a right hand with the thumb pointing in the direction of the current flow; Figure 15.2). Given the thousands of pyramidal cells that are all aligned in palisade fashion orthogonally to the cortical surface, both these generated electrical potentials and magnetic fields summate into ones large enough to be measured by sensors on the scalp or slightly above. When amplified and displayed as signals over time these are identified as EEG and MEG, respectively. FIGURE 15.2. Bottom right: Current flow down a neuron creates a magnetic field around it by the “right-hand rule”—that is, the magnetic field encircles the neuron like the fingers of a right hand with the thumb pointing in the direction of the current flow. Top: The magnetic fields from an activated population of palisaded pyramidal cells summate to produce a larger field that exits the head (efflux maximum) and reenters it (influx maximum). Source: Reproduced with permission from Ebersole JS, Husain AM, Nordli DR. Current Practice of Clinical Electroencephalography. Wolters Kluwer Health; 2014. 380THE EVOLUTION OF CLINICAL MEG EEG measures the electrical potentials on the scalp from electrodes placed in a geometric array. Looking for the “phase reversal” became the key in a simplistic type of source localization that remains popular today. Somewhat forgotten is the fact that EEG in a bipolar montage shows only the differences in potential of adjacent electrodes and not the real amplitude of the signal recorded by each. Clinicians began to think that the phase reversal defined the location of the brain source, and not just the most negative part of any EEG field that this chain of electrodes happened to cross. Only in limited circumstances is the source of any brain electrical activity directly beneath its negative field maximum, identified by a phase reversal using a bipolar montage. The location and strength of both the negative and positive field maxima of an EEG field is required to define its source. FIGURE 15.3. A tangential source, depicted by the red arrow, produces a typical dipolar magnetic field with efflux and influx maxima. This field is detected by pickup coils (A) and amplified by a superconducting quantum interference device (Squid; B). Both are immersed in liquid helium (C) in a Dewar. Source: Reproduced with permission from Ebersole JS, Husain AM, Nordli DR. Current Practice of Clinical Electroencephalography. Wolters Kluwer Health; 2014. 381MEG is a relatively new technique for visualizing brain activity. MEG measurement requires special sensors because the brain’s magnetic fields are extremely small. Both pick up coils that served as flux transformers and the attached superconducting quantum interference devices (SQUIDs), needed to sense MEG signals, require immersion in liquid helium to become superconducting (Figure 15.3). In addition, the entire recording setup and patient need to be housed in a special shielded room that attenuates interfering environmental magnetic fields. From the outset, MEG evolved along different lines than did EEG. Although MEG could be displayed as traces, similar to EEG, it was the measurement of the three-dimensional (3-D) MEG fields around the head that was foremost for source localization. In addition, interpretation was not simply based on traces or even fields, but dependent on the calculation of putative brain sources by means of biophysical models, the most common of which was the single equivalent current dipole (sECD). There was a recognition from the beginning that the generators of MEG produced dipolar magnetic fields, namely ones with both influx and efflux maxima. Furthermore, it was known that the field source lies between the two maxima and not under either one. Finally, the dipole source model was typically co-registered with the patient’s MRI because the calculation of the model provided 3-D coordinates for doing so. It soon became clear that the visualization of a possible source of abnormal brain activity in a patient’s own MRI could be much more revealing than a verbal interpretation in rather vague descriptive terms. EEG fields are also dipolar and they can be interpolated from multiple electrodes as MEG does from multiple sensors. The source of an EEG signal is likewise not necessarily directly underneath the negative field maximum, but rather somewhere between the two maxima, depending upon the strength of each maximum. EEG fields are now analyzed just like MEG fields and modeled to estimate the 3-D location of the cortical generator. Similarly, these estimations can be superimposed upon the patient’s brain MRI. In fact, it has now been shown that EEG source modeling is complementary to MEG source modeling. Both have strengths that offset the weaknesses of the other. Both together provide the most accurate and complete functional localization of brain activity that we can offer patients. EEG VOLTAGE TOPOGRAPHY The major phase of most epileptic discharges is surface negative, that is, the superficial cortical laminae are the site of depolarization (active sink) while the deep laminae are a passive source. The palisaded pyramidal cells of the neocortex not only provide the substrate for EEG because of dipole field summation, it is also the net orientation of these cells and their location within the brain that determine the EEG field orientation and magnitude, which in turn is expressed on the scalp as the location and strengths of the negative and positive field maxima. If the net orientation of the pyramidal cell aggregate is radial, that is, orthogonal to that of the overlying skull/scalp, a higher amplitude negative field maximum will be directly above the source on the ipsilateral side of the head, while a lesser positive maximum will be on the opposite side of the head (Figure 15.4). A 3-D line between the two maxima will go through the center of the head. In this case and in this case only will the generator of the EEG potential be directly below the negative field maximum, that is, below the phase reversal in a traditional bipolar EEG montage. FIGURE 15.4. Example cortical EEG sources 1 to 4 are depicted in a schematic head/brain coronal cross section. Typical pyramidal cell orientations are shown for each source. Resultant scalp voltage field topography for superficial laminae depolarization is displayed above each source as isopotential lines of field strength (speckled = negative, clear = positive). Typical EEG dipoles modeling these sources are depicted beneath each source. Note that simple and more complex cortical convexity sources (2 and 3, respectively) produce radial fields with negative maxima directly above them and positive maxima contralaterally. Radial EEG dipoles deep to the cortex model these sources. Interhemispheric source 1 and temporal base source 4 produce tangential fields with negative and positive maxima displaced to either side of the source and essentially no potential directly above them. Tangential EEG dipoles deep to the cortex model these sources. Source: Reproduced with permission from Ebersole JS, Husain AM, Nordli DR. Current Practice of Clinical Electroencephalography. Wolters Kluwer Health; 2014. Collections of pyramidal cells in most sulci and fissures and on certain gyral surfaces have a net orientation that is tangential, that is, parallel to the locally overlying skull/skin curvature. The EEG fields produced are distinctly different in that the negative field maximum is projected to one side of the generator area and the positive maximum to the other, while no potential is noted directly above it. Often the maxima are both on the same side of the head. The source of this type of EEG field, like that of MEG, is between the two maxima (Figure 15.4). Clearly in the case of a tangential field, the classic EEG phase reversal would be misleading as to its underlying source. 383Between these two extremes are a whole host of cortical generators that produce fields whose orientations are oblique, that is, somewhere between radial and tangential, in which case the negative and positive field maxima are displaced to each side of the source to a variable degree. One should also note that sulci produce sizeable tangential EEG fields only when one side of the sulcus is activated. When both sides are similarly active their fields cancel (see Figure 15.4, source 3). Although the gyri of convexity cortex are likely to produce radial fields and deep fissures and sulci to produce tangential fields, certain gyral crowns also produce tangential fields because of their orientation relative to skull/scalp. These include orbito-, mesial, and interhemispheric frontal cortex, temporal tip, base, and superior plane cortex, occipital base, and interhemispheric cortex. Because of their training, most electroencephalographers look for phase reversals as localizing features in EEG. Accordingly, radial fields are correctly interpreted, whereas tangential fields may be misinterpreted. However, modern digital EEG software provides many methods to detect tangential EEG fields. These include displaying the EEG traces in a common average reference montage that emphasizes the positive as well as negative maxima and shows a more realistic, rather than comparative, field amplitude. Voltage topographic maps clearly show both maxima of a tangential field. Finally, computer-based source modeling is available to visualize likely source orientations and locations in 3-D. EEG dipole source models do suffer from one major problem because the EEG is attenuated and smeared by the overlying skull/scalp. Accordingly, a larger generator area is necessary to create a detectable field due to attenuation during volume conduction of the signal. Both lead to an EEG field of larger proportion than its corresponding MEG field. Since a dipole is a point-like theoretical source, typically it must be deep to the actual generator cortex in order for it to mimic this larger field (see Figure 15.6). Therefore, one must interpret an EEG dipole model. Although an EEG dipole orientation is likely to be true to its generator, one cannot rely on its location to be accurate in its depth. MEG MAGNETIC TOPOGRAPHY The magnetic field generated around each pyramidal cell, reflecting current flow through it, encircles the neuron in the right-hand rule direction (i.e., it is orthogonal to the long axis of the neuron). Thus, the summed magnetic field of a group of neurons is orthogonal to the net orientation of the neuronal population. The field exits the head (efflux) in one location and reenters the head (influx) in another location some distance away (Figure 15.3). The deeper the source the greater the distance between the efflux and influx maxima. Note that the pyramidal cells themselves must be parallel or nearly so to the skull surface for this to happen. This is the same net cellular orientation that would produce a tangential EEG field. If the cellular population that is discharging has a net orientation that is radial to the skull, the magnetic fields do not exit the skull, but simply spread parallel to it. Accordingly, cellular populations with an orientation that produce radial EEG fields do not produce recognizable MEG fields. MEG must be generated by populations of cells that have themselves a tangential orientation or nearly so. The same parts of the cortex that produce tangential EEG fields produce MEG fields, namely sulci, fissures, and tangentially oriented gyral cortex. However, just like EEG, if both sides of a sulcus are equally activated, no net magnetic field results. Unlike EEG, magnetic fields are not perturbed by overlying skull and scalp; they pass through these as though they were not present. There is no attenuation or smearing, so 384smaller cortical areas are needed to produce a recognizable magnetic field. MEG fields thus tend to be more discrete and their field maxima closer in separation than their correlate tangential EEG fields. Because EEG field orientation is the same as generator pyramidal cells, while MEG field orientation is orthogonal, the surface field maxima of both are orthogonal in location to each other (see Figure 15.6). MEG dipole source localizations tend to be more accurate than EEG because MEG sources can be smaller, fields from the same source are more compact, and they are not distorted by skull/scalp. This gives MEG a distinct advantage over EEG, if the cellular population in question produces a tangential field. As the orientation of the generator population becomes less tangential and more radial, the MEG field generated becomes progressively smaller in amplitude compared to the background noise of uncorrelated brain activity, that is, the signal to noise ratio (S/N) lessens. At some point, probably around an orientation of 45 degrees from tangential, source localization by MEG ceases to be better than by EEG. It becomes obvious that using both techniques provides the best overall solution—MEG for more tangential sources, EEG for more radial sources. Smaller cortical sources that produce purely tangential fields are not appreciated by EEG; cortical sources that produce purely radial fields are not appreciated by MEG. MEG/EEG SOURCE MODELING Electro-magnetic brain signals provide crucial information regarding their brain source. Mapping the fields of these signals reveals complementary dipolar patterns of EEG and MEG that are suited for source analysis. Consequently, MEG and EEG can be source localization tools for depicting the origin of normal and abnormal brain activity. For MEG, a set of four clinical practice guidelines provides important information and minimal recommendations for clinical MEG practitioners (See Bagić et al., 2011a, b, c and Burgess et al., 2011 in Additional Resources). There are no endorsed clinical practice guidelines for EEG source localization at this time. For the estimation of the origin of brain activity with MEG or EEG, two models are needed: (a) a head model (also called volume conductor model) and (b) a source model. Head models simulate important physical and anatomical characteristics of the human head. The most commonly used ones are spherical (single or multiple spheres) or boundary element method (BEM) models. The latter requires the patient’s individual MRI (MPRAGE or MULTI-ECHO sequences) visualizing the entire head with 1 mm slice thickness. Brain tissue, cerebrospinal fluid, dura mater, skull, and scalp have in common very low and almost identical magnetic susceptibility characteristics. Hence a very simple spherical or a single compartment (brain) BEM model, that is straightforward to create, is sufficient for the purpose of magnetic source estimation. For EEG source modeling, volume conductor models are more demanding. The aforementioned concentric tissues of the human head have very different conductivity properties with a ratio between them of up to approximately 1:80. The skull has the lowest conductivity of all. Considering these differences, a more complex head model, such as the multi-compartment BEM model, is often required, particularly for the basal portions of brain/skull that are less spherical. Source modeling calculates solutions to the origin and temporal dynamics of electro-magnetic brain activity, based on various mathematical algorithms. It can sometimes be difficult to summarize the richness of MEG or EEG data with one or a few dipolar point sources. 385Accordingly, a variety of source localization algorithms have been developed beyond the sECD in an attempt to explain more fully the complexity of clinical data. These include scanning methods, beamformers, current density, entropy, and connectivity methods. All source localization methods have inherent strengths and limitations to consider. Most of these latter methods use a regularized grid of dipoles for modeling purposes. While the sECD shows only the best-fitted single point source, other methods that optimize for different parameters might display the best 5%, 10%, or more of their dipole solutions, hence appearing more like a “distributed source.” These can be visually more appealing, however, their maps of spike activity do not show the actual cortical extent of the focus, rather only a mathematical possibility. Noninvasive MEG and EEG data do not provide enough information to solve the problem of source extent. Based on the “Okada constant” of approximately 1 (one) nAm of current produced per mm2 of cortex (See Murakami & Okada, 2015, in Additional Resources), the minimal extent of a source can be loosely inferred. For example, a MEG spike with a dipole strength of 200 nAm should have a minimal area of not less than 200 mm2, a square with 14 mm side length. The source area for such a spike could be and probably is much larger. In this chapter we will focus on the sECD model, which has been the most well-studied and validated tool for localizing the epileptogenic focus. Clinical MEG practice guidelines recommend always using the sECD initially and, if needed, augmenting it with additional source models. However, when other source modeling algorithms are employed, they should always be compared to the sECD result of the same data. It is critical for the reading clinician to provide a clear and concise interpretation of the findings, which results in a cohesive clinical report. Using too many different source models can make a report impenetrable for the referring physician, who is usually not familiar with their strengths and limitations. SCIENCE BEHIND DIPOLE INTERPRETATION MEG and EEG source modeling can directly estimate the location, orientation, and strength of current dipoles. In MEG evoked field studies of sensory-motor activity, it is typical to see ECDs with a source strength (or dipole moment) of approximately 20 nAm, and when modeling interictal spikes, a much stronger source of 100 to 500 nAm is often noted. Dipole modeling can implement numerical acceptance ranges for a set of specific source parameters, as well as statistical testing against a baseline. The source parameters in question for MEG include the confidence volume of the dipole solution (not larger than 1,000–1,500 mm3), the source strength (100–500 nAm), and a goodness of fit of the solution of usually 90% or higher (when using a region of interest that encloses 60–80 MEG channels). Statistically, a reduced Chi2 test should provide results between 1 and 2. The confidence volume is often displayed as an ellipsoid around the best fitting dipole (see Figures 15.6–15.14). A source anywhere within this ellipsoid is also possible given the signal/noise ratio of the data. The smaller the confidence volume the smaller the uncertainty of the source estimate. Such objective approaches are very helpful in rejecting individual transients that are not model-worthy from the analysis and interpretation process. This rigorous approach frequently yields focal clusters of MEG sources with parallel orientations and similar direction, because statistical outliers are not considered. 386DATA REVIEW AND SOURCE MODELING INDIVIDUAL SPIKES OR AVERAGES Raw MEG data, like that of EEG, are reviewed as traces of magnetic field strength and polarity over time as recorded by sensors. Since MEG is reference free, there are no bipolar or referential montages per se. Data from the array of sensors in the MEG helmet are typically displayed in some anatomical arrangement to appreciate more easily local and regional brain activity. MEG epileptic spikes appear similar to EEG spikes, but they may be sharper than EEG spikes, because only tangential field components that may be more coherent are recorded. The primary and secondary phases of a MEG spike, reflecting neuronal depolarization and repolarization, are often equally sharp for the same reason. The later phases of an EEG spike are progressively less sharp, because they are generated by a more complex combination of radial and tangential activity, which inevitably results in less synchrony and thus waveform sharpness. Since MEG spike fields are bipolar with both an efflux and influx maxima, MEG trace displays typically show phase reversals, both toward and away. Accordingly, skills obtained in reading bipolar EEG are actually quite useful in reviewing raw MEG data for spikes. However, just as in EEG, there are many sharp MEG transients that are not epileptic spikes. The best way to determine whether or not a given transient is possibly a spike, and not an artifact, is to visualize its magnetic field topography. A genuine MEG spike will have a well-formed and compact dipolar magnetic field. As discussed earlier, the location and orientation of the field maxima are a reflection of the location and orientation of their cortical source. Source localization can thus be approximated simply by inspecting a spike’s field. For MEG, the source will lie between the two maxima and its orientation can be obtained by the right-hand rule. However, it is routine in clinical MEG to go one step beyond field mapping and submit either individual spikes or spike averages to source modeling, such as the sECD. FIGURE 15.5. Dipole models of individual right temporal spikes are shown at left. Note the differences in location and orientation among the cluster and the large confidence volume (red ellipse) of the red dipole. A dipole model of the averaged spike is shown at right. Note the small confidence volume of this dipole given the improved signal to noise of the averaged spike.
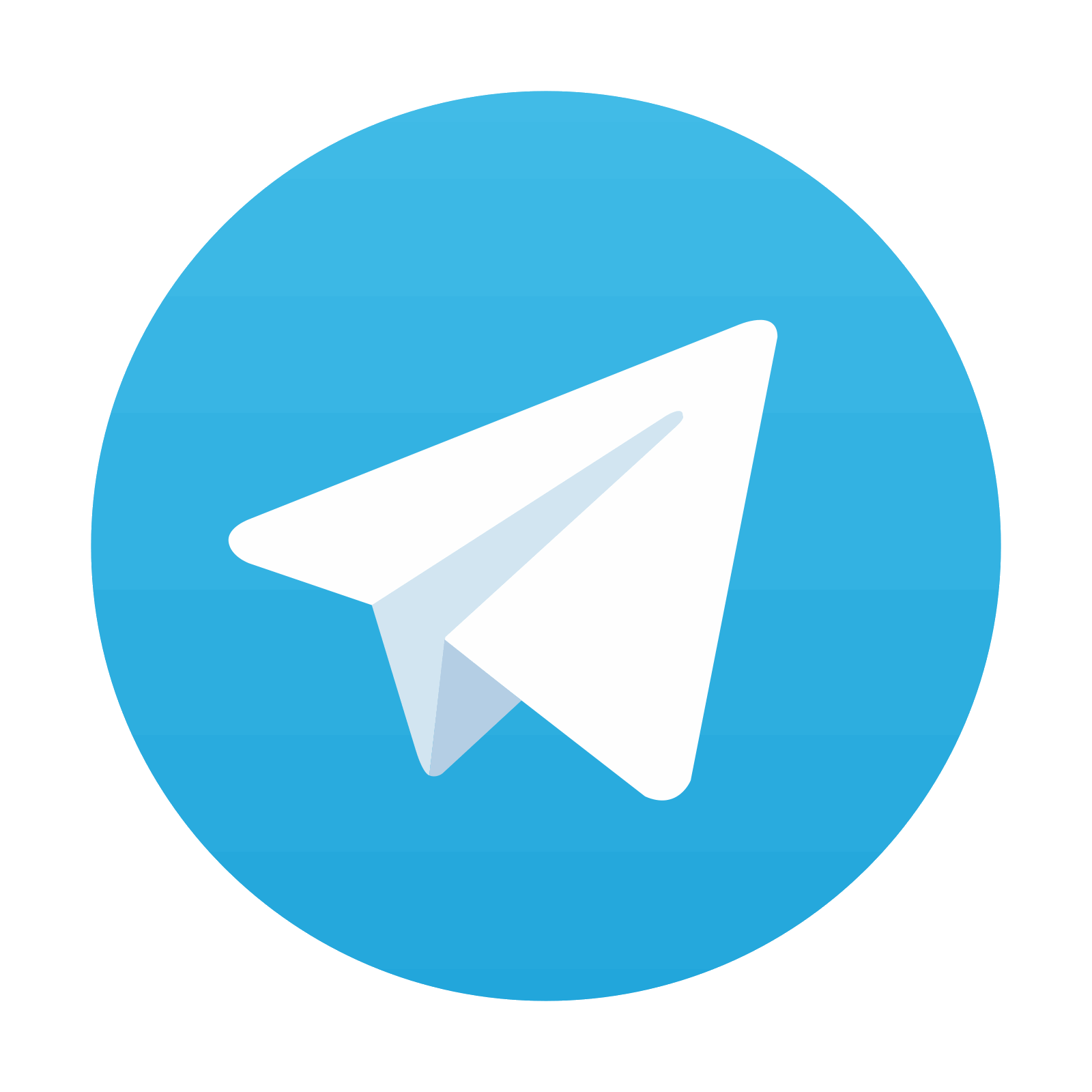
Stay updated, free articles. Join our Telegram channel
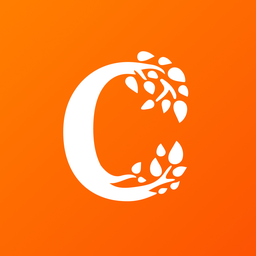
Full access? Get Clinical Tree
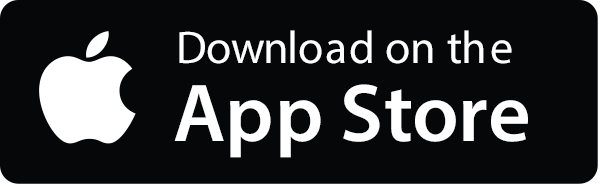
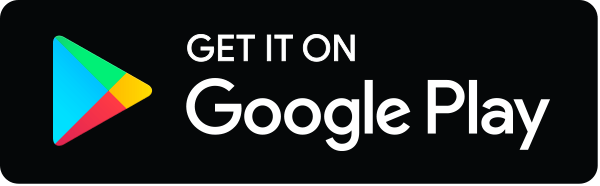