A 45-year-old man with no significant past medical history presents to the intensive care unit (ICU) after a motor vehicle collision. Upon presentation to the Emergency Department (ED), he was endotracheally intubated for airway protection, with a Glasgow Coma Scale (GCS) of 7 (E1V2M4). Intubation was accomplished without any incidence of hypotension or hypoxia; however, the patient was actively vomiting at presentation and vomitus was noted at his vocal cords during direct laryngoscopy. His trauma work up included computed tomography (CT) scans of the head, cervical spine, chest, abdomen, and pelvis, as well as a chest radiograph. This demonstrated bifrontal contusions on CT and suspected diffuse axonal injury (DAI). No other injuries were discovered. He presents to your ICU early in the night with the following settings on the mechanical ventilator: assist control/volume-targeted mode (AC/VC), tidal volume, 650 cc; positive end-expiratory pressure (PEEP), 5 cm H2O; respiratory rate, 14; and a fraction of inspired oxygen (Fio2) of 0.50. On these settings, you note that his peak inspiratory pressure is 29 cm H2O, and his inspiratory plateau pressure is 25 cm H2O. The patient’s vital signs are as follows: heart rate, 115; blood pressure, 120/80 mm Hg; respiratory rate, 14; oxygen saturation, 92%; and temperature, 99.5°F. Also, as you review the graphics package on the ventilator, you note smooth waveforms, with no evidence of intrinsic PEEP and no evidence of dysynchrony. As you receive the report from the Emergency Medicine physician, you note the concerns for aspiration, and you review his chest radiograph and chest CT, which both show a suggestion of aspiration to the bilateral lower lobes. You are happy with his current status, but you wonder how his head injury and potential lung injury will progress. As you settle in for an interesting call night, you notice that his inspiratory plateau pressure is now 35 cm H2O and his Fio2 is 0.80.
Regardless of the indication for endotracheal intubation and initiation of mechanical ventilation, a mechanical ventilator in and of itself, does not treat much. However, it does have great potential for harm. Effective mechanical ventilation should prioritize limitation of ventilator-associated lung injury (VALI) almost as much as oxygenation, and maintenance of normal carbon dioxide levels should be a lower priority in the grand majority of patients.1 Keen knowledge of basic and advanced principles can allow the clinician to use the mechanical ventilator for its maximum therapeutic potential and minimize iatrogenic injury, while the process that led to respiratory failure is allowed to heal. It cannot be stressed enough that knowledge of cardiopulmonary physiology, heart-lung interactions, and the effects of mechanical ventilator settings on cerebral oxygenation and blood flow, will allow the clinician flexibility when titrating support to an individual patient at the bedside. Although the clinician should be intimately familiar with guidelines and large clinical trials, they are only as good as the response a particular patient has to a chosen therapy.
Mechanical ventilation is instituted for a number of reasons (Table 42-1).2 Most commonly, these indications are a combination of a failure to adequately oxygenate, ventilate, or meet the metabolic demands of a physiologically stressed patient. Type I respiratory failure is hypoxemic respiratory failure, defined as a partial pressure of oxygen in arterial blood (Pao2) < 60 mm Hg. Type II respiratory failure is hypercarbic respiratory failure, defined as a partial pressure of carbon dioxide in arterial blood (Paco2) > 50 mm Hg.3 Mechanical ventilation may also be instituted to maintain normal blood pH, decrease work of breathing, and assist left ventricular function in the setting of acute decompensated heart failure or for airway protection in the setting of toxic overdose, traumatic brain injury (TBI), or any other significant acute CNS illnesses.
Physiologic Reason | Clinical Assessment | Normal Value | Abnormal Value Suggesting Need for Mechanical Ventilation |
---|---|---|---|
Hypoxemia | A-a gradient Pao2/Fio2 ratio Sao2 | 25-65 350-400 98% | > 350 < 300 < 90% |
Hypercarbia/inadequate alveolar ventilation | Paco2 | 35-45 mm Hg | Acute increase from patient’s baseline pH < 7.15 Mental status decline |
Oxygen delivery/oxygen consumption imbalance | Elevated lactate Decreased mixed venous oxygen saturation | 2.2 mg/dL 70% | > 4 mg/dL despite adequate resuscitation < 70% despite adequate acute resuscitation |
Increased work of breathing | Minute ventilation Dead space | 5-10 L/min n/a | > 15-20 L/min n/a |
Inspiratory muscle weakness | NIP VC | 80-100 cm H2O 60-75 cc/kg | < 20-30 < 15-20 |
Acute decompensated heart failure | Jugular venous distention Pulmonary edema Decreased EF | Clinical judgment combined with the above factors |
The breath generated by a mechanical ventilator can be separated into four phases: triggering, inspiration, cycling, and expiration.2,4
Triggering represents the change from expiration to inspiration and occurs either because of a drop in circuit pressure or flow (when patient triggered) or as a result of elapsed time. Sensitivity refers to a preset threshold of pressure or flow. When this is reached, a mechanical breath is delivered. This can be manipulated by the clinician, and is usually set to a –1 to –2 cm H2O. In the setting of pressure triggering, airway pressure is reduced (by patient effort) in the proximal circuit, the expiratory valve closes, and the patient receives a breath. In the setting of flow triggering, the patient’s inspiratory effort induces a disruption of the constant flow in the ventilator inspiratory circuit. This change in flow signals the expiratory valve to close and the ventilator to deliver the next breath.
For inspiration, pressurized gas is channeled from the ventilator to the patient after the exhalation valve closes. This phase can be controlled (or targeted; see below) based on volume or pressure. For example, AC/VC is volume control/targeted and AC/PC is pressure control/targeted. Choice of control variable is largely the discretion of the clinician, as either can be manipulated to achieve set goals. It should be noted though, that in volume-targeted ventilation, excessive airway pressures can arise secondary to worsening pulmonary mechanics. In this situation, the pressure alarm will cause a pressure limit to cycle to expiration. In pressure-targeted ventilation, minute ventilation is not guaranteed. It is a function of the compliance and resistance of the respiratory system. The clinician therefore should monitor these physiologic changes closely to avoid untoward changes in either airway pressure or Paco2 levels.
The pattern of inspiratory flow can be square, decelerating, or sinusoidal (Figure 42-1). Square inspiratory flow pattern results in a rapid rise to a preset level (set by the clinician) followed by constant flow until cycling occurs. Decelerating flow results in a rapid rise to a maximum level followed by a gradual decrease until cycling. This is secondary to the decrease in pressure difference (Δ pressure) between the ventilator and patient as inspiration progresses during pressure targeted ventilation. All pressure targeted breaths are of the decelerating flow pattern. Sinusoidal flow pattern most closely represents normal physiologic breathing. It results in flow that gradually increases then decreases during inspiration. The choice of inspiratory flow pattern should be based on patient characteristics, and a few common clinical scenarios should be familiar to the clinician. Square flow over time results in a shorter inspiratory time (I time), and therefore longer expiratory time (E time). For this reason, it is usually preferred in patients with obstructive physiology (chronic obstructive pulmonary disease [COPD] or asthma).5,6 It is also usually tolerated better in patients with demand for high minute ventilation, such as severe metabolic acidosis or elevated intracranial pressure (ICP). Under this situation, there is potential for dysynchrony to occur during the progression of the inspiratory phase if decelerating flow is chosen. The tradeoff is higher peak airway pressures with a square wave form. Decelerating flow results in a longer I time and a better distribution of flow. With pulmonary pathophysiology involving heterogenous distribution of injury (ARDS as the prototype example), decelerating flow is likely the best choice. The length of inspiration depends on several factors. In pressure-targeted modes of ventilation, the clinician directly controls the I time and I:E ratio. In volume targeted ventilation, the I time is a function of set tidal volume (VT) and flow rate (VT/inspiratory flow rate).
Cycling is the transition from inspiration to expiration. It can occur because of decrease in flow, elapsed time, or delivered volume. Flow-cycled breaths are pressure support (PS) breaths. When a predetermined decrement in flow occurs, inspiration is terminated and the patient cycles to expiration. This is typically set at 25% of initial flow, but can be manipulated based on different clinical conditions. For example, if a large leak occurs (bronchopleural fistula or ventilator circuit leak), it may be wise to set the cycle at a higher percentage of initial flow to terminate inspiration at the appropriate time. It should be noted that for safety reasons, PS can be cycled due to excessive pressures or elapsed time. In time-cycled breaths, inspiration is terminated after a preset interval, regardless of whether preset pressure has been achieved. Pressure targeted ventilation is time cycled. In volume-cycled breaths, the clinician has selected the targeted tidal volume. Volume is delivered until that volume is reached. Airway pressure is directly dependent on the tidal volume and the mechanical characteristics of the patient’s respiratory system. For safety reasons, volume-cycled breaths will be pressure cycled if airway pressures exceed the pressure alarm limit. Under this situation, the delivered tidal volume will not reach the set volume target, unless the pressure alarm limit is increased (the clinician should exercise caution with this strategy).
Flow from the ventilator is stopped, the exhalation valve opens, and gas is allowed out from the lungs. This occurs passively. Inspiratory flow should cease prior to expiration. If this does not occur, the patient will develop auto PEEP or intrinsic PEEP (iPEEP). This is most commonly seen in patients with expiratory flow limitation (COPD or asthma) or higher I:E ratios (Table 42-2).
Trigger | Target/Limit | Cycle | |
---|---|---|---|
Volume control | Time | Flow/volume | Volume |
Volume assist | Patient effort | Flow/volume | Volume |
Pressure control | Time | Pressure | Time |
Pressure assist | Patient effort | Pressure | Time |
Pressure support | Patient effort | Pressure | Flow |
A ventilator mode describes the set of breath characteristics delivered to the patient, patient-ventilator interactions and includes such things as control variable/target, phase variables, and mandatory vs spontaneous breaths.2,7 Two general approaches exist and involve either setting volume/flow as the control variable or pressure. From there, a variety of specific and complicated combinations can be derived. The choice of ventilator mode is largely at the discretion of the clinician. Pressure targeted vs volume/flow targeted have more in common than not, can achieve similar frequency and minute ventilation goals, and can equally be employed to limit VALI. For this reason, clinician familiarity, ICU, and institutional practice patterns determine to a large extent the mode that is employed. Also, data showing definitive improvement in clinically relevant outcomes when comparing one mode vs another is lacking. Patient physiology and knowledge of mode differences will allow greater flexibility to titrate safe mechanical ventilation based on bedside evaluation, clinical scenario, and response to therapy. When confronted with a patient who is difficult to oxygenate and whose compliance is dynamically worsening, pressure-targeted modes of ventilation may be most effective. Setting a pressure target identifies a priority of minimizing VALI over guaranteed minute ventilation, and the decelerating inspiratory flow pattern also allows for better gas distribution into heterogeneously injured lung units. Also, the clinician has more direct control of the I:E ratio, therefore allowing easier manipulation of these variables to maximize mean airway pressure relative to peak and plateau airway pressures, which is beneficial for oxygenation.
In controlled mechanical ventilation (CMV), the great majority of the patient-ventilator interaction that occurs is from the ventilator to the patient and not vice versa. For example, it is time triggered and is limited and cycled by the ventilator. Although this mode of mechanical ventilation is no longer an available selection, this is what is essentially achieved when a patient is not interacting because of heavy sedation or neuromuscular blockade. The term control in this instance should not be confused with the control variable or target, as described below. Assisted breaths can either be volume or pressure targeted (eg, AC/PC or AC/VC). With these types of breaths, the clinician sets the target (tidal volume or pressure) and a set respiratory rate. The patient can then trigger the ventilator and receive the set target each time, more frequently than the set rate on the ventilator. In this instance, the ventilator is doing work, specifically on the patient, and therefore assisting the breath after the patient triggers/initiates it. Assisted breaths also encompass intermittent mandatory ventilation, where the ventilator delivers a set number of breaths, but the patient can trigger spontaneous breaths as well, as well as pressure support ventilation (PSV), where the patient triggers all the breaths and the delivered volume is determined by the set pressure, patient effort, and mechanical characteristics of the respiratory system.
Controlled breaths vs spontaneously triggered breaths are undoubtedly different with respect to respiratory muscle function and viability, gas exchange properties, lung expansion, and hemodynamics.8 A fully controlled breath must fully expand the lungs and chest wall without inspiratory muscle assistance. Spontaneous breathing also serves to maintain venous return by lowering pleural pressure and therefore right atrial pressure.9 Diaphragmatic movement is more pronounced ventrally in the supine patient without spontaneous breathing. This favors atelectasis in dorsal lung units, and a ventral distribution of ventilation, resulting in increased dead space ventrally and shunt dorsally.10 In patients with vigorous respiratory muscle activity, a switch to fully controlled ventilation may be beneficial. In this setting, oxygen consumption (Vo2) can decrease substantially, and by removing expiratory muscle contraction, end-expiratory lung volume can be preserved, which improves oxygenation.8 The preservation of spontaneous breathing is of more benefit than harm in the great majority of patients. However, in the setting of very high demand, such as severe, acute, early acute respiratory distress syndrome (ARDS), there may be a role for fully controlled breaths, but this should be titrated to the individual patient.11-13
For a breath to occur, whether it be spontaneous or by positive pressure, a pressure gradient must exist from the airway opening to the alveoli.2,4,7,14 The volumes delivered and pressures generated largely depend on the mechanical properties of the respiratory system: the lungs and chest wall, as well as the abdomen. Each of these components has mechanical properties that determine the overall behavior of the respiratory system. Although the respiratory system can be quite complex, the main variables of interest are really only pressure, flow, and volume. Specifically, the ventilator must generate a pressure to cause flow and therefore increase lung volume.14 The pressure required to do this reflects a combination of the pressures to inflate the lung and chest wall. This can be illustrated by the equation of motion:14,15 Muscle pressure + ventilator pressure = (elastance × volume) + (resistance × flow).
The equation of motion illustrates several basic principles. A ventilator can only directly control one of the three variables at a time (pressure, volume, or flow). Despite its complexity a ventilator is simply a machine controlling one of these three variables (control variables).2,4,7 They are also referred commonly as the “target” and are the variables that the ventilator holds constant despite changing respiratory system mechanics. For example, in pressure control ventilation, pressure is the control variable or target and is held constant, whereas flow and tidal volume will vary based on the patient’s respiratory mechanics. Control in this instance should not be confused with a controlled vs an assisted breath, as above.
Compliance describes the ability or tendency of the respiratory system to expand in response to a delivered pressure and volume.14,16 Simplistically, this is ΔVolume /ΔPressure, and the compliance of the respiratory system (CRS) is ΔV/ΔPalveolar. Elastance is the inverse of compliance, or the ratio of pressure change to volume change, and describes the tendency to recoil. Resistance describes the impedance to airflow through the respiratory system, or the ratio of pressure change to flow change.
A pressure-volume (PV) curve (Figure 42-2) demonstrates key features of respiratory system mechanics.17,18 The lower (LIP) and upper inflection points (UIP) reveal areas of reduced compliance secondary to low and high volumes, respectively (compliance is volume dependent). As de-recruited alveoli are opened up, the lower inflection point transitions into the steeper (most compliant) portion of the PV curve. This is theoretically the physiologically most sound and safe portion to ventilate the patient, though where this lies in an individual patient can be difficult to elucidate. At higher tidal volumes, the upper inflection point represents a transition to over distention, risk for barotrauma, and reduced compliance. This graphically represents a foundation for the concept of PEEP setting and VALI (below).19 The PV curve also demonstrates hysteresis: greater compliance during expiration vs inspiration. This reflects the fact that recruited alveoli are more compliant than recruiting or unrecruited alveoli and provides the foundation for the concept of recruitment maneuvers in PEEP setting, as well as advanced modes of mechanical ventilation, such as airway pressure release ventilation (APRV) and high-frequency oscillatory ventilation (HFOV). The normal lung and chest wall are remarkably compliant (200 cc/cm H2O), and the normal compliance of the respiratory system is about 100 cc/cm H2O (lung compliance and chest wall compliance added in series).14 The severely injured lung may have compliance as low as 20 cc/cm H2O or even lower. Unfortunately, CRS that is displayed by the mechanical ventilator has to be interpreted with caution. This number represents a weighted average of many million alveolar units; in pathophysiologic states exhibiting heterogenous injury distribution (eg, ARDS), compliance, volume distribution, and potential for VALI vary greatly.20,21 Resistance to airflow is determined by artificial and natural airways, as well as the rate and pattern of flow. Similar to compliance, resistance varies with volume, but also flow, and differs between inspiration and expiration.
The three most common airway pressures displayed for monitoring on a mechanical ventilator are: peak airway pressure (Ppeak), inspiratory plateau pressure (PPL), and mean airway pressure (Pmean) (Figure 42-3).15 Ppeak reflects CRS as well as resistance. Elevated Ppeak can lead to barotrauma, increased pulmonary vascular resistance, and right heart dysfunction, as well as decreased venous return.22 Under no-flow conditions (inspiratory hold maneuver on the ventilator), resistance is eliminated, and this pressure reflects PPL. The distending force of the lung is the transalveolar pressure: alveolar pressure minus pleural pressure.14,23 In the setting of elevated pleural pressure, such as elevated intraabdominal pressure or reduced chest wall compliance, transalveolar pressure may be quite low; therefore, PPL in the “safe” range may lead to significant underventilation.23 Currently, without the ability to directly measure pleural pressure, the PPL is the best reflection of transalveolar pressure, the distending pressure of an alveolus, and is an important reflection of potential for VALI. Of note, pleural pressure can be measured indirectly, via an esophageal balloon, and this pressure can be used in the calculation of transalveolar pressure.24 Pmean is the average airway pressure over the respiratory cycle. Elevating the mean airway pressure should be viewed as a strategy to improve recruitment and oxygenation, with the goal being elevation to a greater extent relative to Ppeak and PPL.25 If a patient has an elevated Ppeak relative to PPL, the problem lies in increased resistance, such as bronchoconstriction or endotracheal tube blockage or kink. If both Ppeak and PPL are elevated, then a compliance problem exists, such as worsening lung injury, pneumothorax, or elevated intraabdominal pressures.
Because of a declining GCS, your recently admitted patient has a repeat head CT revealing expansion of the hemorrhagic contusions in the bifrontal lobes, as well as worsening edema and intraventricular hemorrhage. The patient now has an external ventricular drain (EVD), parenchymal intracranial pressure (ICP) monitor, and a brain tissue oxygen monitor placed. You have yet to manipulate his ventilator settings, and the high pressure alarm repeatedly is heard. A repeat chest radiograph now shows progression of his aspiration to diffuse, bilateral alveolar infiltrates consistent with ARDS. His airway pressures are now in the mid-40s and his oxygen saturation is 90% on a Fio2 of 1.0. The arterial blood gas (ABG) shows the following: 7.27/50/58. Your fears are confirmed and you now realize that your patient is now becoming one of the most challenging cases seen in the ICU: the patient with severe TBI who is difficult to oxygenate and ventilate. You think quickly how not only to manipulate the ventilator to oxygenate and ventilate this patient, but how to do so safely, with a strategy to limit VALI and ICP, while promoting brain oxygenation and cerebral blood flow (CBF).
Knowledge of the general concepts of mechanical ventilation is of little use without knowledge of the patient at the bedside and defining the goals of therapy for that individual patient. With all critically ill patients, goals can be defined as oxygenation, limitation of VALI (perhaps just underneath oxygenation in order of importance), and ventilation. However, in the neurologically injured patient, we must add limitation of secondary brain injury.26 This includes hypoxia, hypotension, prolonged periods of hypercapnia or hypocapnia, and elevated ICP. Manipulation of the mechanical ventilator can directly affect all of these.
Mechanical ventilation is common in the management of the neurologically injured patient.27-29 Unfortunately, the direct generalization of many high-quality clinical trials is difficult in this patient population, as TBI is often an exclusion to trial enrollment.30 To add to the complexity, protective ventilation strategies and the management of TBI have often been viewed as having competing and discordant therapeutic targets, such as permissive hypercapnia, and PEEP setting to ameliorate VALI.27 To this end, the ventilator must be adjusted to (1) improve brain oxygenation and (2) improve CBF. At a very minimum, it must be adjusted to not harm cerebral oxygenation and blood flow. They are intimately linked, and inevitable overlap exists, but will be discussed separately to provide a balanced approach with seemingly competing and potentially conflicting therapeutic strategies in the neurologically injured patient requiring evolving mechanical ventilation requirements.
Oxygenation should be the primary goal in any mechanically ventilated patient, especially the neurologically injured. Under normal conditions, alveolar ventilation is nearly equal to cardiac output, producing an overall ventilation/perfusion (V/Q) ratio close to unity.31 While the exact causes of hypoxemia are innumerable, only a few physiologic mechanisms explain arterial hypoxemia (Table 42-3).3 In the critically ill, mismatch between alveolar ventilation and perfusion and right to left shunt, are by far the most common mechanisms. Diffusion is rarely the cause of hypoxia, unless severely impaired.
Mechanism | Therapeutic Strategy for Reversal |
---|---|
Alveolar hypoventilation | Endotracheal intubation |
Decreased FIO2 | Supplemental oxygen. Try to maintain FLO2 ≤ 0.60 |
Diffusion abnormality/increased DLCO | Reversal of pathologic process |
V/Qc mismatch | Alveolar recruitment, PEEP setting, reversal of hypoxic pulmonary vasoconstriction, inhaled nitric oxide, limit intrinsic PEEP |
Shunt | Alveolar recruitment, PEEP setting, prone positioning, closure of patent foramen ovale |
Decreased oxygenation of SvO2 | Decrease oxygen consumption, hemodynamic support, increase cardiac output |
Oxygenation can be assessed several ways.32 A noninvasive pulse oximeter allows assessment of arterial oxygen saturation. Arterial blood gas analysis gives us the partial pressure of arterial oxygen (Pao2). Perhaps greater indicators for an oxygenation problem, calculation of the alveolar-arterial oxygenation (A-a) gradient, the Pao2:Fio2 ratio, or oxygenation index (OI) gives the clinician greater insight into the extent of oxygenation failure.
Normal arterial oxygen saturation (Sao2) and Pao2 are > 95% and > 80 mm Hg, respectively. In the critically ill patient, these goals can sometimes be unattainable. Setting oxygenation goals in the critically ill can be largely empiric, but patient factors and available data should be considered. The majority of high quality acute respiratory distress syndrome ARDS trials have allowed for a Sao2 of 88% and a Pao2 of 55 mm Hg.30,33-36 It should be noted that at these levels, the patient is most certainly on the steep portion of the oxyhemoglobin dissociation curve and is in danger of a quick decline in arterial oxygenation with any decline in cardiopulmonary physiology, circuit disruption, or daily patient care activities. Hypoxemia not only will decrease cerebral oxygen delivery, but also result in cerebral vasodilation, therefore raising ICP. The Brain Trauma Foundation recommends keeping arterial oxygenation above Pao2 of 60 mm Hg. Other guidelines, including the UK Transfer guidelines for brain injury (97.5 mm Hg) recommend even higher oxygenation targets.37,38 Given the well-known association of hypoxemia with worse outcome.26,39-42 as well as the consideration of guideline recommendations, the neurologically injured patient should have these goals raised. Hypoxia should be avoided at all costs, and the ARDS net targets are likely too low in this patient population. A target of Pao2 of 60% and Sao2 of 92% at a minimum is likely reasonable, and these goals should be revisited based on individual patient physiology.
Increasing the fraction of inspire oxygen (Fio2) is perhaps the simplest and quickest way to improve arterial oxygenation and should be done immediately in the setting of life-threatening hypoxia. Unfortunately, simply increasing Fio2 may be the least physiologic way to improve oxygenation, from a pulmonary mechanics standpoint, and prolonged administration of high levels of oxygen has consequences.
Lung tissue is exposed to the highest concentrations of oxygen in the body. In animal and human studies, high fractions of inspired oxygen can cause lung injury, ranging from mild subjective symptoms to diffuse alveolar damage that is histologically indistinguishable from ARDS.43,44 Exposure to elevated Fio2 can reduce lung volumes secondary to absorptive atelectasis, increase intrapulmonary right to left shunt, and directly injure pulmonary parenchyma. The onset and rate of development are likely directly related to level of oxygen administration, as well as duration.
Hypoxia is known to cause secondary brain injury and the delivery of Fio2 1.0 has been shown to increase brain tissue oxygenation.45 Typically, the partial pressure of brain oxygen tension (Pbto2) measured by a brain tissue oxygen probe improves almost immediately when Fio2 is increased.45 However, the administration of high levels of oxygen should be cautioned against. Despite some studies demonstrating reduced lactate levels and reduced lactate to pyruvate ratios, other studies have not reproduced these findings, and no good clinical studies demonstrate benefit of normobaric hyperoxia.46-49 In fact, recent data show worse outcome associated with hyperoxia in cardiac arrest, stroke, and TBI.50-53 In addition, systemic hyperoxia has not been shown to improve cerebral metabolic rate or functional outcome.54 Given these negative findings, as well as the well-known toxicity of hyperoxia, administration of excessive levels of oxygen should be discouraged in favor of better ventilator management to maximize recruitment, PEEP effects, and the compliant portion of the PV curve. When Fio2 exceeds 0.60, the clinician should manipulate the ventilator settings to improve oxygenation.
PEEP maintains airway pressure above atmospheric pressure throughout the respiratory cycle by pressurization of the ventilator circuit. A full review of the topic is beyond the scope of this chapter, as the published literature on PEEP is extensive. However, the clinician should be aware of the pathophysiology pertinent to PEEP setting, advantages and side effects, approaches to PEEP setting, and the use of PEEP in the neurologically injured patient.
In patients with hypoxia due to atelectasis, alveolar edema and/or volume loss (these cause most type I respiratory failure), PEEP serves to restore functional residual capacity (FRC), reduce intrapulmonary shunt, shift ventilation to a more compliant portion of the PV curve, and prevent end-expiratory volume loss (derecruitment).55 PEEP recruits previously nonaerated lung tissue and homogenizes regional distribution of tidal ventilation. The net effect on gas exchange reflects the balance between recruitment and overdistention. In the setting of obstructive physiology or expiratory flow limitation, PEEP serves less to improve oxygenation, but more to improve patient-ventilator synchrony and triggering (below).5,6,56
PEEP is not without side effects. Given the heterogenous distribution of lung injury in ARDS, PEEP can over distend more compliant lung units, contributing to VALI.57 If PEEP leads to over distention, it can augment dead space, increase pulmonary vascular resistance, and cause right heart dysfunction. It can also decrease venous return, and in the setting of volume depletion, decrease cardiac output.
The optimal way to set PEEP is widely debated and controversial, as is the optimal level of PEEP to use.34-36,58-62 The literature reveals that PEEP has been set to the following: maximal compliance, to a level just exceeding the lower inflection point on a PV curve, which is the lowest PEEP to achieve adequate oxygenation, or to a PEEP-Fio2 combination table.34,60,63,64 There is no widely accepted method to enhance clinical outcome. Before implementing any PEEP setting strategy, an assessment of the risks and benefits, but most importantly, the potential to recruit alveolar units should be ascertained. Patients with primary pulmonary disorders, such as pneumonia or pulmonary contusions, usually have lung units with much less recruitability compared with an extra-pulmonary etiology of hypoxia, such as sepsis.65 Villar et al set PEEP at 2 cm H2O above the lower inflection point and demonstrated a mortality decrease from 53.3% to 32%.60 Using higher PEEP, Ranieri et al demonstrated attenuation in cytokine response, suggesting decreased inflammatory signaling with higher PEEP.62 These studies were done with simultaneous decrease in tidal volumes, however. The ALVEOLI trial was done to determine the isolated benefit of higher levels of PEEP in patients with ARDS. Despite improvement in oxygenation, the higher PEEP group showed no improvement in clinically relevant outcomes.34 In two recently published trials, high PEEP was set based on a “lung open ventilation” protocol along with recruitment maneuvers and was adjusted as high as possible to not exceed PPL 28 to 30 cm H2O. Both of these trials demonstrated improved oxygenation, but no mortality benefit.35,36 Another physiologically attractive alternative is PEEP setting under the guidance of an esophageal balloon. This balloon is placed similarly to a nasogastric tube and is used to estimate pleural pressure, in order to calculate transpulmonary pressure. Recent data show an improvement in oxygenation and compliance, but again no improvement in clinically relevant outcomes.24 Data has shown wide variability with respect to lung recruitment and PEEP effect on lung recruitment. As such, individualizing PEEP setting is likely the most prudent approach.
PEEP can be set with or without recruitment maneuvers, with the aim being to open collapsed alveoli that may need higher, sustained inflation pressures to open. Clinical characteristics, such as etiology of ARDS, can help assess potential for recruitment, but likely the easiest way to assess potential for alveolar recruitment is with a recruitment maneuver. After recruitment, alveoli are more compliant and easier to keep open. Recruitment maneuvers have been applied as sustained inflation pressures (30-40 s at 35-50 cm H2O), because pressure controlled breaths at increased PEEP levels, or as intermittent sighs.35,59,63,66-69 They are generally very effective at improving oxygenation; however, they should be followed by an incremental increase in PEEP, or the effects are only transient. In general, recruitment maneuvers are safe and tolerated well, but should likely be limited to patients early in the course of lung injury (more recruitability), with bilateral lung disease and no evidence of hypovolemia.
PEEP can adversely affect ICP and cerebral perfusion pressure (CPP), and setting the PEEP in patients with neurologic injury has been a controversial topic.26,27 By increasing intrathoracic pressure, and therefore juxtacardiac pressure, right atrial pressure can increase. This can impede venous outflow from the brain and lead to elevated ICP. Also, if PEEP decreases cardiac output, this can lead to hypotension and reduced CBF. These effects are usually less significant in patients with reduced lung compliance. Also, if PEEP leads to overdistention, as opposed to alveolar recruitment, an increase in dead space can lead to elevated Paco2 levels. Elevated ICP and reduced CPP have historically been viewed as (relative) contraindications to the use of increasing levels of PEEP. This has lead to the observation of a lower threshold to simply increase the Fio2 to combat hypoxia in this patient population. Fortunately, the literature demonstrates that PEEP is well tolerated in TBI patients.26,27,70-72 Early studies show that PEEP causes only modest, clinically insignificant changes in ICP.73-76 In fact, PEEP set lower than ICP typically does not result in an increase in ICP, and if PEEP achieves alveolar recruitment, it can reduce ICP. Even in the setting of normal lung compliance (and therefore greater transmission of pressure to the intravascular space), PEEP has been shown to not significantly increase ICP. Furthermore, if PEEP results in alveolar recruitment, brain oxygenation may improve as a result.77 Therefore, TBI and elevated ICP should not be considered contraindications to PEEP setting in patients who need alveolar recruitment. The data and physiology dictate that the benefits likely outweigh the risks in a majority of patients.
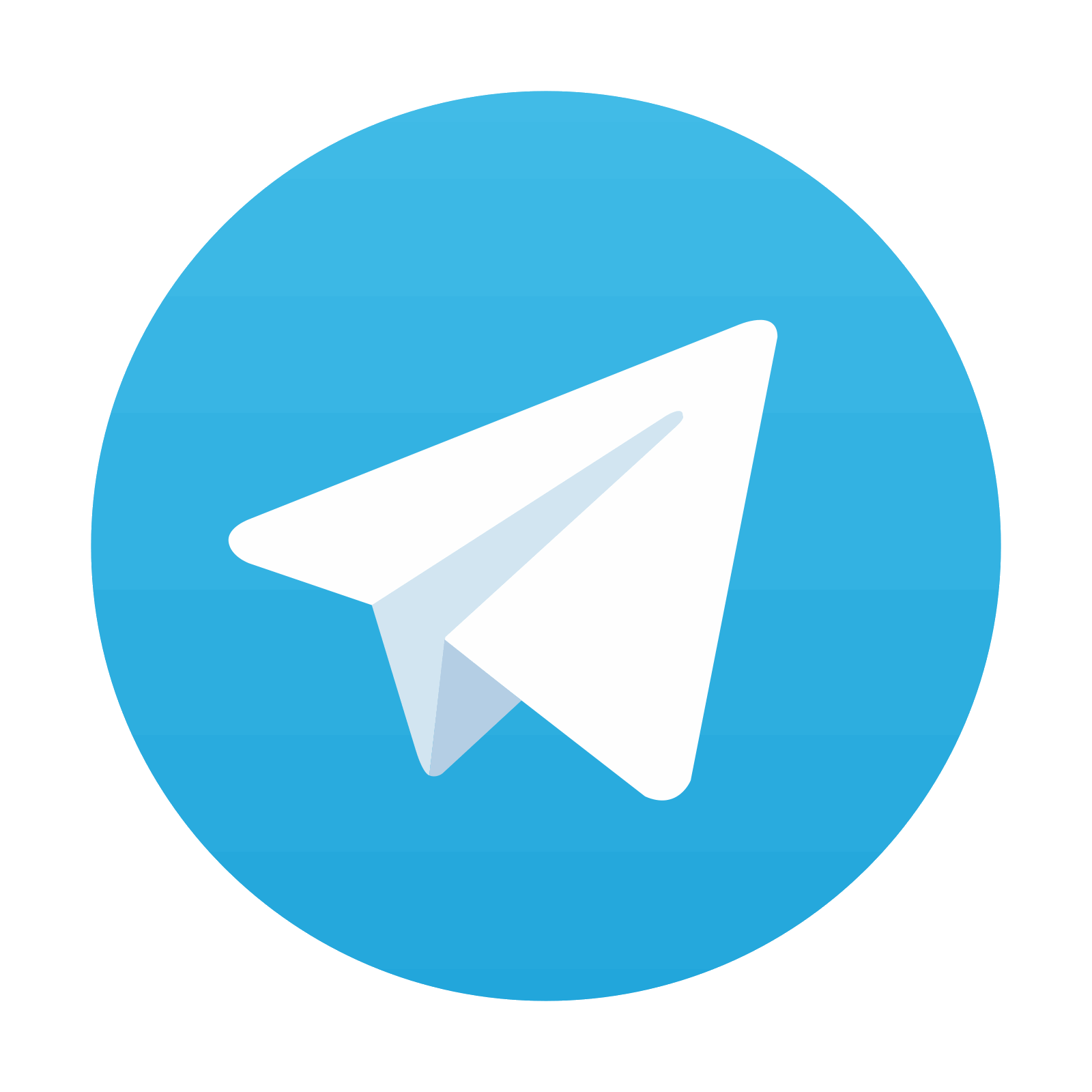
Stay updated, free articles. Join our Telegram channel
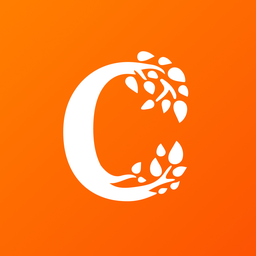
Full access? Get Clinical Tree
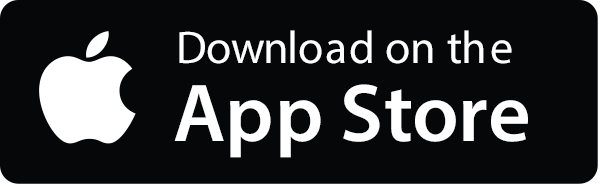
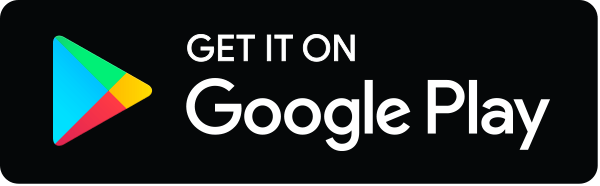