Fig. 5.1
Potential mechanisms of axonal injury in progressive MS
5.4.1 Inflammation
Inflammatory mediators have long been implicated in the pathophysiology of MS [35]. A variety of cellular and soluble factors cause inflammatory damage to myelin and oligodendrocytes and a number of putative mechanisms have been described [36]. Immune mechanisms involved in myelin injury include both cellular, with T cells directed against myelin and oligodendrocyte components with subsequent macrophage and microglial recruitment; and humoral immunity, with secretion of antibodies by B cells and subsequent complement involvement in opsonization and phagocytosis of myelin debris [37]. Common mechanisms are likely to operate in the pathogenesis of inflammatory axonal injury, although the science of myelin injury derived from animal models of central nervous system inflammation is more advanced. Axonal injury may be mediated by cellular attack and/or the effect of soluble factors, including cytokines and free radicals. These will be reviewed in some detail.
5.4.1.1 Cellular Injury
Although the initial immunological cascade in MS is thought to be a T-cell-dependent process, multiple cellular effectors are involved in tissue damage and specifically axonal injury. T lymphocytes play an important role in the generation and propagation of immune changes in MS through autoimmune activation via as yet unknown antigen stimulation [38]. In EAE, CD4+ T cells polarized to a Th (T-helper; and specifically Th1) phenotype induce much of the immunological damage and indeed these cells are prominent within active MS lesions [39]. Dysregulated Th1 responses have been implicated in much of the organ-specific autoimmunity of MS through actions of cytokines, such as γIFN, and through the activation of macrophages and microglia. MHC class 1-restricted, CD8+ T cells are also prominent in active MS lesions and Th17 cells (producing IL-17) are also likely to play an important role in MS [40]. T-reg (FOXp3+) cells are involved in the process of tolerance and immunosuppression and other cells such as invariant natural killer (NK) cells, CD56+ NK cells, and possibly stem cells have roles in immunological injury and immunomodulation [41].
With regards to cell-mediated axonal injury in MS, CD8+ T cells and microglia are currently thought to be the major cell types involved. Interestingly, class 1 MHC molecules, which are required for antigen presentation to CD8+ T cells, are rarely expressed in electrically active neurons but are expressed during prolonged electrical silence and when neurons are exposed to γIFN [42]. Neurites expressing class 1 MHC molecules are then susceptible to damage from CD8+ T cells [43]. Thus, a combination of the inflammatory milieu and the electrical state of the neuron may combine to induce axon injury. CD8+ T cells are prominent in active MS plaques and their numbers have been shown to correlate with axonal injury within lesions [17, 18]. The relative contribution of CD8+ T cells versus CD4+ T cells to axonal injury in MS is unknown, but common mechanisms involving cytokine release (see below) are implicated.
Microglia-mediated axonal injury has been studied in EAE and in vitro. Levels of acute axonal transection correlate well with microglial numbers in human postmortem tissue [14]. Microglia effect tissue injury via a number of mechanisms, with the major mechanism of action thought to be through production of reactive oxygen and nitrogen species (see below). The contribution of microglia to chronic axonal injury is unknown.
Whether primary antibody-mediated axonal injury occurs in MS is not currently clear. The study of auto-antibodies in CNS inflammatory disorders has recently been rejuvenated by the discovery of anti-aquaporin-4 antibodies in neuromyelitis optica which are directed against astrocyte water channels [44]. Using a proteomics-based approach, antibodies to neurofascin were identified in a subset of patients with MS [45]. Neurofascin is a neuronal protein which is concentrated at the node of Ranvier of myelinated fibers. Co-transferring anti-neurofascin antibodies with oligodendrocyte glycoprotein-specific encephalitogenic T cells into rodents led to specific antibody targeting of the nodes of Ranvier with subsequent axonal injury. Interestingly, changes in neurofascin staining patterns have been documented in human postmortem tissue from patients with MS, which is associated with axonal loss [46]. Similarly, antibodies against neurofilaments have been detected in spinal fluid samples of patients with MS [47, 48]. Determining whether the presence of these antibodies represents primary and specific antibody-mediated targeting of axons or whether they occur in response to widespread tissue injury with exposure of previously sequestered antigens, remains a challenge. It is likely, however, that many of the antibody responses are epiphenomena.
5.4.1.2 Reactive Oxygen and Nitrogen Species
In vitro and in vivo studies have revealed potential mechanisms by which reactive oxygen and reactive nitrogen species (ROS/RNS) may damage central nervous system axons. Nitric oxide (NO), which serves many physiological functions within the nervous system, has been implicated as a likely mediator of axonal injury, and at sites of central nervous system inflammation (both MS itself and experimental models of MS), high concentrations of NO are found [49]. Pathological studies have also shown activity of the enzyme responsible for production of NO (inducible nitric oxide synthetase (iNOS)) within MS lesions [50, 51]. Microglia produce NO, which is a major mechanism by which these cells induce axonal injury [52, 53]. In vivo application of nitric oxide donors to rat dorsal root axons causes degeneration when electrically stimulated [54]. Interestingly, blockade of the electrical activity by sodium channel blockers or inhibitors of sodium/calcium exchange process may attenuate axon destruction [55]. A potential explanation for these observations is that NO inhibits mitochondrial respiration, causing failure of sodium/potassium ATPase, which, in turn, leads to increased levels of sodium within the axon, causing a reversal in the sodium/calcium exchanger, allowing excess levels of calcium to mediate axonal degeneration [49]. In vitro studies have shown NO causes dose-dependent neurotoxicity via a number of intracellular pathways, ultimately leading to inhibition of mitochondrial respiration and cell death [56–59]. NO also has specific axonotoxic effects in vitro, and high concentrations of NO reduce neurofilament phosphorylation levels within axons, leading to axonal destruction [60, 61]. A number of other reactive oxygen and reactive nitrogen species (ROS/RNS) may also be important in the pathogenesis of axonal injury in MS [62].
5.4.1.3 Cytokines
Cytokine-mediated tissue damage has also been extensively studied in relation to MS and indeed there have been trials of anti-cytokine therapies for the disease. Two cytokines in particular have attracted attention – γIFN and TNFα. The cytokine profiles classically associated with Th1 cells, γIFN, TNFα, and IL-2, are found in acute lesions and these cytokines (as well as mRNA transcripts of these cytokines) can be detected in tissue and CSF derived from patients with MS [63, 64]. The deleterious effect of γIFN on axonal survival in neuroinflammatory conditions may be via activation of microglia (see above) [58]. Circulating TNFα levels are raised in MS relapses and the role of TNFα in oligodendrocyte injury and demyelination has been extensively studied [65, 66]. While much evidence exists for a pro-inflammatory function for TNFα, the function of TNFα in MS is likely to be complex. For instance, some reports have demonstrated a likely anti-inflammatory effect of TNFα [67]. The probable explanation for the variability in effects in animal models is the pleiotrophic effect of TNFα on different cell types, acting through different receptors. TNFα acts through TNFR1 or TNFR2 and the majority of existing knowledge concerning the actions of TNFα are based on studies of TNFR1 (the so-called death receptor) signaling. The function of TNFR2 in the brain is poorly understood and some studies have suggested that TNFα activity via TNFR1 or TNFR2 may exert a neuroprotective role, potentially through mobilization of anti-oxidant molecules [68, 69]. The complexity of the role of TNFα in MS was further highlighted by the disappointing trials of TNFα-blocking agents in the disease, which caused exacerbation of disease activity [70, 71]. Understanding the precise effects of TNFα and other cytokines on axons in the context of MS remains a priority.
Glutamate has attracted attention as a mediator of white matter excitotoxicity [124]. Changes in glutamate activity have been reported in multiple sclerosis tissue [125]. Glutamate mediates both myelin and axonal damage in a variety of in vitro and in vivo models [126]. Again, calcium overload secondary to reversal of the sodium/calcium exchanger seems a possible final common pathway mediating glutamate toxicity in white matter.
Whether inflammation plays any protective role in neuroinflammatory conditions is an important question, since the rationale for almost all therapies for multiple sclerosis has been to reduce inflammation. Trying to elucidate any potential beneficial effects of inflammation may help to tailor anti-inflammatory therapies to be more specific. The inherent repair that occurs in the early stages of MS (remission) suggests a powerful beneficial effect of certain mediators involved in the immune response. Inflammatory cells, notably T lymphocytes, produce growth factors and cytokines which may be beneficial in promoting repair [72]. Specifically, brain-derived neurotrophic factor (BDNF) immunoreactivity within lymphocytes in multiple sclerosis lesions has been demonstrated [73]. Intriguingly, following alemtuzumab therapy, lymphocytes may show increased expression of a number of neurotrophic factors, including BDNF, platelet-derived growth factor (PDGF), and ciliary neurotrophic factor (CNTF), which may be a partial explanation of some of the improvements seen in disability following therapy with the drug [74]. In experimental models of demyelination, inflammation may encourage remyelination which, in turn, may have beneficial protective effects on the axon [75]. The recognition that immune cells may contribute to neuroprotection implies nonspecific immunosuppression may not always be beneficial at all stages of the disease.
5.4.2 Chronic Demyelination
As discussed, both treatment trials and epidemiological data have suggested that inflammation alone is unlikely to be responsible for all of the progressive disability seen at later stages of the disease and therefore other “noninflammatory mechanisms” must be postulated. The respective contributions to disease progression of inflammatory and noninflammatory mechanisms are unknown. Indeed, they may vary as the disease becomes more chronic and inflammation may contribute more early in the disease course. Chronic demyelination of normally myelinated axons has been suggested as a possible mechanism for non-inflammatory axon injury and a number of experimental models have shown that myelin and oligodendrocytes have trophic properties towards axons.
CNS myelin contains a number of proteins and glycoproteins, which are involved in the development and maintenance of the axon–myelin unit. These include proteolipid protein (PLP) and DM20 (an alternate-spliced isoform), myelin basic protein (MBP), myelin-associated glycoprotein (MAG), myelin–oligodendrocyte glycoprotein (MOG), and 2′,3′-cyclic nucleotide 3′-phosphodiesterase (CNPase). A number of myelin mutants have been generated experimentally (or exist naturally), which have demonstrated the importance of myelin to axon development. For example, shiverer mice have an absence of compact CNS myelin (caused by a deletion in the MBP gene) and exhibit multiple axonal abnormalities, including an increase in slow axonal transport, alterations in microtubule density and number, and altered neurofilament assembly and phosphorylation [76–78].
Similarly, myelin components are thought to be involved in long-term axon maintenance. Studies have shown that PLP may have a major role in axonal maintenance, since mice lacking the plp gene are able to synthesize large amounts of myelin, yet show late-onset axonal degeneration with “ovoid” formation and impairment of axonal transport mechanisms [79, 80]. Indeed, axons may become dependent on oligodendrocyte support sometime after myelination has been completed, as non-myelinated axons within optic nerves from chimeric females (which show patches of normally myelinated and non-myelinated axons) never develop axonal swellings over the expected time course. Mice which are engineered to express the peripheral myelin component P0 instead of PLP within the CNS show a structure and periodicity of myelin which resembles peripheral myelin, yet had severe disability and degeneration of myelinated axons, indicating a specific central neuroprotective role of CNS myelin [81]. Other components of myelin also provide trophic support to axons. For instance, mice lacking CNPase have morphologically normal myelin, but show delayed axonal swelling and degeneration [82]. A problem with the study of myelin mutants is that axons are never exposed to “normal” myelin and thus, it may be argued, may develop abnormal axon–glial interactions, and axon degeneration in these models may not be relevant to diseases in which myelin contains a full complement of components. A recent study of a noninflammatory model of demyelination has shown that chronic loss of axons occurs even in the absence of a significant primary immunological event and in the presence of myelin containing all the necessary components of compact CNS myelin (Fig. 5.2) [83]. Importantly, replacement of myelin has been shown to protect axons from chronic axon loss induced by demyelination [84].


Fig. 5.2
Progressive demyelination is associated with axonal injury in the taiep rat: Proteolipid protein (PLP) immunohistochemistry in sections of cerebellum from wild-type (a, b) and taiep rats (c, d) at 3 months (a, c) and 12 months (b, d). Non-phosphorylated neurofilament (SMI32) immunohistochemistry in sections of cerebellum from wild-type (e, f) and taiep rats (g, h) at 3 months (e, g) and 12 months (f, h). Scale bar in (d) represents 1,000 μm for (a–d); bar in (h) represents 100 μm for (e–h) (Figure reproduced with permission from Wilkins et al. [83])
The relevance of myelin components to axon maintenance in humans may be studied in related white matter disorders. Pelizaeus–Merzbacher disease (PMD) is caused by disturbances in the function of the PLP gene [85]. While most cases of PMD are caused by PLP duplication, some are caused by a null mutation in the PLP gene, which is associated with a milder clinical phenotype and a relative preservation of myelin [86]. Axonal abnormalities occur in these patients, even in areas where myelin levels appear normal, and MRS imaging data in PMD has shown a decrease in NAA levels in the CNS when compared to control, consistent with decrease in axonal densities [87]. These observations indicate the importance of myelin to maintenance of human axons within the CNS.
Oligodendrocytes may influence axon survival and development in ways other than through the production of myelin. Oligodendrocytes release a variety of growth factors which may influence neuronal survival and axonal phenotype, including insulin-like growth factor type-1 (IGF-1), nerve growth factor (NGF), brain-derived neurotrophic factor (BDNF), and glial cell line-derived neurotrophic factor (GDNF) [88–90]. Oligodendrocyte-derived GDNF specifically protects axons in cell culture models via increases in neurofilament phosphorylation states [60, 91]. Whether oligodendrocyte-derived factors provide significant trophic support for axons in vivo under normal physiological settings is unclear, but axonal degeneration in MS could potentially be mediated, at least in part, by chronic oligodendrocyte depletion.
5.4.3 Other Chronic Axonal Changes in MS Which May Contribute to Axonal Loss
A number of other pathological changes occur in axons which may predispose to chronic degeneration. Many of these occur as a consequence of disturbance of the axon–glial subunit. For instance, alterations in ion channel composition and distribution occur along the length of demyelinated axons. Clustering of sodium channels (particularly Nav1.6) at the node of Ranvier is a physiological principle that underpins saltatory conduction and demyelination causes redistribution of ion channels [92]. Sodium channel clustering is dependent on oligodendrocyte signaling since md (myelin deficient) mice (which lack oligodendrocytes but not astrocytes) show almost no sodium channel clustering along axons at postnatal day 16, an age at which the phenomenon is prominent in wild-type mice [93, 94]. Reduction in clustering and expression of Nav1.6 along the length of demyelinated axons occurs in EAE [92]. This serves as a physiological adaptive response to preserve propagation of the action potential, but may also have chronic deleterious effects on axonal stability. Expression of Nav1.6 along the length of demyelinated axons is accompanied by increased expression of the Na+/Ca2+ exchanger [95]. Increased levels of sodium within axons may cause reversal in the sodium/calcium exchanger which allows excess levels of calcium to mediate axonal degeneration, as discussed above [55]. Furthermore, electrical activity in axons exposed to inflammatory mediators may further dysregulate ion flow within axons, leading to degeneration [54]. These observations have formed the basis for trials of sodium channel blockers in MS [96].
Disrupted mitochondrial function may contribute to axonal degeneration in MS. Trying to determine whether specific mitochondrial abnormalities occur in MS has been the subject of a number of studies. Interest was first generated in the field with the observation that an MS-like illness occurs in some patients with Leber’s Hereditary optic neuropathy (LHON), which is caused by mutations in mitochondrial DNA [97]. The energy requirements of demyelinated axons are greatly increased due to the diffuse spreading of voltage-gated sodium channels along the axolemma and the inefficiency of action potential propagation [98]. Furthermore, increase in intra-axonal sodium ion levels mentioned above may be caused by reduced mitochondrial ATP production, leading to failure of the Na+/K+ ATPase pump [92]. The most obvious way in which mitochondrial dysfunction might link into axonal degeneration in MS is through blocking of respiratory chain enzymes by ROS/RNS. Substances such as NO, produced as part of the inflammatory cascade in the disease, cause damage to mitochondrial DNA and decrease oxidative phosphorylation [99]. Changes in mitochondrial complex I, III, and IV occur within axons and neurons of MS tissue, either representing an adaptive response or potentially a pathogenic mechanism [100, 101]. Recently, it has been shown that nuclear export of histone deacetylase 1 (HDAC1) into the cytoplasm is necessary for the onset of axonal damage in an experimental model of MS [102]. Cytosolic HDAC1 may impair mitochondrial transport mechanisms within axons and thus contribute to reduced energy production. Further research into mitochondrial abnormalities in MS axons is ongoing.
5.4.4 Normal-Appearing White Matter and Grey Matter
Axonal changes also occur in so-called “normal-appearing white matter” (NAWM) albeit at much reduced levels compared to acute lesions [14, 15]. NAWM contains, by definition, normal levels of myelination, so chronic demyelination or inflammation occurring in the local vicinity of the area of axon damage cannot be the cause. It is possible that axonal changes in NAWM may result from a Wallerian degenerative process. That is to say, if an axon traverses a segment of inflammation or demyelination, changes may occur not only locally but at areas proximal or distal to the plaque. A putative mechanism for this is that disrupted axonal transport mechanisms within the plaque leads on to axonal dysfunction throughout its length, although this remains to be proven [103]. An alternative to this is that diffuse axonopathy occurs independently of inflammatory demyelination as a primary neurodegenerative process, but the evidence for this is sparse [104].
Another consideration is injury to neuronal cell bodies and axons within the grey matter. Grey matter pathology, predominantly occurring in the cerebral cortex is now a well recognized phenomenon in multiple sclerosis [105]. The degree of grey matter damage correlates well with physical disability and particularly cognitive impairment in MS [10]. Indeed, rate of disease progression appears to correlate with the severity of cortical inflammation [106]. Injury to the cell body of a neuron may lead to axonal dystrophy and loss by a number of processes, including inflammation. Interestingly in MS, cortical inflammation appears different to white matter inflammation. Cortical lesions have reduced levels of inflammatory cellular infiltrate, and pure intra-cortical lesions (i.e., ones that do not straddle the grey-white matter interface) typically have low levels of inflammation [107]. The presence of meningeal inflammation and ectopic B-cell follicles adjacent to cortical lesions has suggested a role for these aberrant B-cell follicles in the pathogenesis of cortical lesions [108]. However, the association between meningeal inflammation and cortical demyelination remains contentious [109]. Interestingly, previous Epstein Barr virus (EBV) infection correlates with the presence of B cells, plasma cells, and ectopic follicles in meningeal infiltrates of patients with MS [108]. The link between EBV and MS pathogenesis has been postulated for some time and EBV remains the strongest candidate as an environmental risk factor for the disease [110]. The work on EBV and B-cell follicles has suggested that viral latency within the CNS of those with MS may contribute to on-going pathology. Further, understanding of the etiology and pathogenesis of cortical lesions and particularly, mechanisms of neuronal cell body injury, will help to further understand disease mechanisms in progressive disease.
5.5 How Does the Understanding of Disease Mechanisms in Progressive MS Inform Future Therapies?
How then might advances in our understanding of the mechanisms of progressive disease translate into the development of new therapies? Broadly speaking, therapies in MS fall into the categories of drug treatments and, currently only in the early preclinical stages, cell-based therapies. Major drug therapies in current use as disease-modifying therapies (DMTs) are discussed elsewhere in this book. In general, the influence of these drugs on the progressive phase of MS has been disappointing. As pointed out above, this may be because of noninflammatory mechanisms of tissue injury occurring at later stages of the disease.
Nonetheless, a major component of axonal injury, particularly in the acute lesion, appears to be inflammation-driven. Anti-inflammatory therapies thus undoubtedly have a role in preventing axonal injury early in the disease course. We know that if anti-inflammatory therapies are instituted after the onset of disease progression, however, their efficacy in preventing subsequent disease progression is poor [32]. However, recent and emerging evidence suggests that early treatment with powerful immunosuppressant drugs such as alemtuzumab, prior to onset of the progressive phase, may reduce progressive disability over time [111]. These observations are significant advances in the treatment of MS and yet several issues remain. Not all patients with MS have relapses and 10–15% have a primary progressive phenotype and therefore would be unsuitable for aggressive immunosuppression. Furthermore, not all patients with MS will go on to develop progressive disease or disability and exposing those patients to potentially toxic drugs would not appear to be in the individual’s best interest. At the present time, the monoclonal antibody therapies (natalizumab and alemtuzumab) are generally restricted to those with severe aggressive disease or those who have failed on other therapies. It may be that as we learn more about the inflammatory process in MS that specific components of axon damage induced by inflammation can be targeted. For instance, understanding more the role of anti-neurofascin antibodies or other cellular effectors in axonal injury may allow targeted immunomodulation [46]. Nitric oxide may also be a potential therapeutic target, although iNOS inhibition may lead to a broad range of cellular responses which are not always beneficial to survival [49]. “Blanket” immunosuppression is at best ineffective in progressive disease, and may possibly hinder intrinsic repair mechanisms, as discussed.
5.5.1 Neuroprotection in MS?
Neuroprotection has long been discussed in the context of chronic neurodegenerative diseases and has recently become a major topic for MS research. MS has its own specific issues which need to be addressed in the development of neuroprotective strategies: the diffuse, rather multifocal nature of the disease; prolonged time phase of disease progression; and difficulties of delivering the treatment to a disease disseminated in time and space within the nervous system.
Neuroprotectants may act in a variety of ways, for example: preventing build-up or action of toxic material within the neuron/axon; directly influencing intracellular signaling pathways within neurons/axons to reverse signaling effects that cause neurotoxicity; or stabilizing axon structure. Classically, inhibition of excitotoxic compounds has been the main thrust of neuroprotectant research for a wide variety of neurological disorders and inhibition of glutamate transmission using riluzole has shown beneficial effects in EAE, an observation that has yet to be systematically replicated in multiple sclerosis [112]. Similarly, the observation that excess intra-axonal sodium results in toxic levels of intra-axonal calcium has been the rationale for trials of sodium channel blockade in MS. Sodium channel blockade using both flecainide and phenytoin have reduced axonal injury in models of multiple sclerosis [55, 113]. Initial trials are underway to test the hypothesis in patients and trial results are beginning to be published [96].
Other drugs may have general neuroprotective effects via a number of signaling pathways. Cannabinoids have rather broad-spectrum effects in experimental models, including modulation of immune responses and direct neuroprotectant properties via specific cannabinoid receptors [114]. Trials are underway to determine the extent to which these compounds are able to impact on disability, and cannabinoids are now clinically available to treat spasticity in MS. Similarly, minocycline, a drug designed initially as an antibiotic, has a pleiotropic mode of action but exhibits direct neuroprotectant properties in vivo and in vitro [115]. Specifically, minocycline increases levels of phosphorylated neurofilament within axons exposed to both inflammatory mediators and withdrawal of trophic support [116]. This leads to improved survival of axons, suggesting its role as a putative therapeutic agent in multiple sclerosis. Again, further trials are underway to investigate the role of the drug in MS therapy [117]. The recent observation showing that nuclear export of HDAC1 is required to induce axonal damage in EAE has raised the possibility that HDAC inhibition may be a further therapeutic option, specifically to protect axons [102]. Other intracellular signaling pathways that may be modulated in neuroinflammatory conditions include the peroxisome proliferator-activated receptor (PPAR) pathways. Oligodendrocyte peroxisomal function appears vital in the reduction of inflammation and in axonal protection in the CNS [118]. The PPAR-α agonist fenofibrate (in current clinical use as a lipid-lowering agent) improves peroxisomal function and protects axons in cell culture models of inflammation [52].
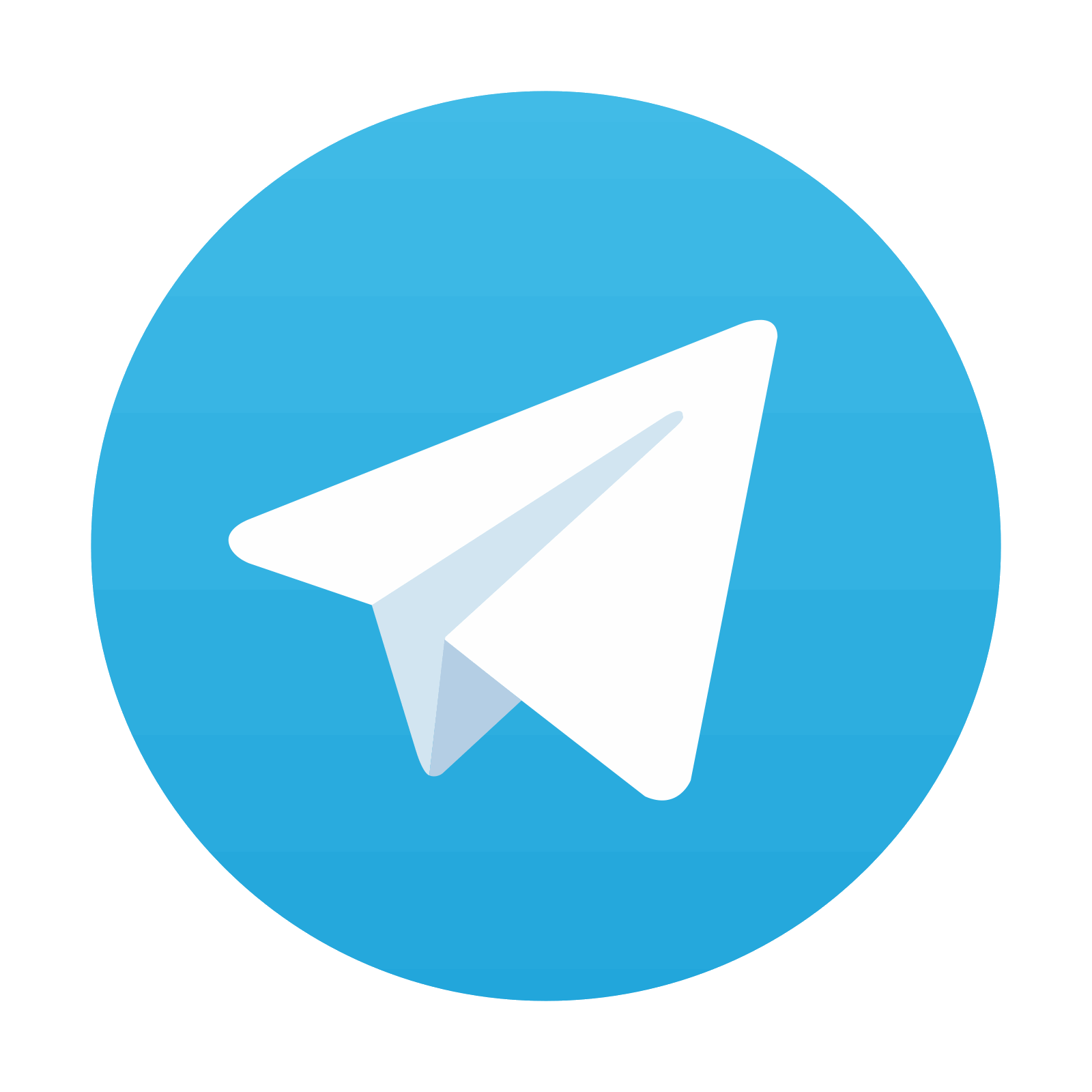
Stay updated, free articles. Join our Telegram channel
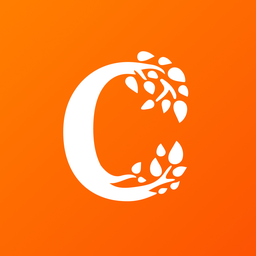
Full access? Get Clinical Tree
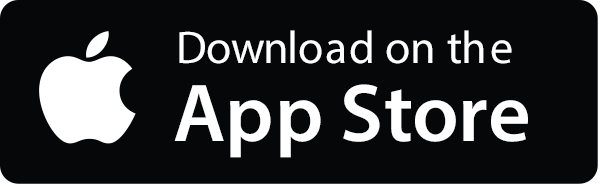
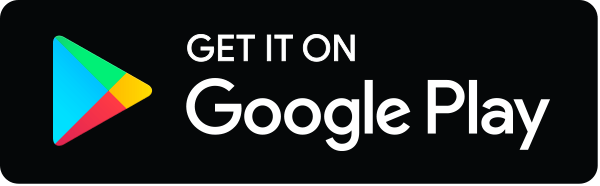