Effective monitoring is critical for neurologically compromised patients, and several techniques are available. One of these tools, cerebral microdialysis (MD), was designed to detect derangements in cerebral metabolism. Although this monitoring device began as a research instrument, favorable results and utility have broadened its clinical applications. Combined with other brain monitoring techniques, MD can be used to estimate cerebral vulnerability, to assess tissue outcome, and possibly to prevent secondary ischemic injury by guiding therapy. This article reviews the literature regarding the past, present, and future uses of MD along with its advantages and disadvantages in the intensive care unit setting.
Key points
- •
Microdialysis is a neuromonitoring technique for direct measurement of cerebral metabolism.
- •
Microdialysis has a role in traumatic brain injury and subarachnoid hemorrhage, and the clinical applications continue to expand.
- •
Microdialysis may be used as a research tool for studying the cerebral effects of medications, pathophysiologic mechanisms, or other cerebral metabolic processes.
Introduction/history
Monitoring the brain after acute injury is central to the practice of neurocritical care and is analogous to hemodynamic monitoring for the cardiovascular system. However, the most widely available clinical monitor, the physical examination, is unavailable in neurologically compromised or sedated patients. Current neuromonitoring techniques have evolved from the study of cerebral physiology and allow for the repeated or continuous measurement of multiple cerebral parameters. These measurements may detect evolving abnormalities and thus guide interventions designed to minimize secondary injury after neurosurgical interventions, traumatic brain injury (TBI), or subarachnoid hemorrhage (SAH). However, no monitor alone can improve patient outcome. Only actions such as decompressive craniectomy for increased intracranial pressure (ICP), based on monitoring data, carry the prospect of improving outcome, and for microdialysis (MD), this aspect requires further studies.
Many neuromonitoring devices attempt to assess changes in cerebral metabolism. This process may be indirectly estimated from global imaging modalities such as xenon computed tomography (CT) and through measuring cerebral oxygen content with jugular venous oxygen saturation (Sjv o 2 ) catheters and cerebral oxygenation (partial pressure of oxygen in brain tissue [Pbt o 2 ]) probes. Direct metabolic substrate measurement in the living human brain currently can only be achieved with cerebral MD catheters or positron emission tomography (PET) scans, which can only be done at great cost and for 1 or 2 time points.
The use of small (approximately 1 mm) semipermiable coaxial and loop membrane tubes within the brain was first described by Bito and colleagues in 1960 and was later refined in 1972 with the advent of the coaxial MD catheter by Delgado and colleagues. In 1986, the first data on neurotransmitter concentrations in the rat brain were reported by Tossman and colleagues.
The first human implantation occurred in 1987 in adipose tissue and was followed in 1990 with the first use in human cerebral tissues. In 1992, the first clinical use of this device was reported, and the creation of commercially available catheters and bedside analysis systems followed shortly. Over time, MD has gained popularity in trauma, stroke, and aneurysmal SAH, and, as more studies verify its usefulness, the indications for use will continue to expand. As of 2012, about 12 centers in North America and about 30 worldwide clinically use brain MD on a regular basis.
Introduction/history
Monitoring the brain after acute injury is central to the practice of neurocritical care and is analogous to hemodynamic monitoring for the cardiovascular system. However, the most widely available clinical monitor, the physical examination, is unavailable in neurologically compromised or sedated patients. Current neuromonitoring techniques have evolved from the study of cerebral physiology and allow for the repeated or continuous measurement of multiple cerebral parameters. These measurements may detect evolving abnormalities and thus guide interventions designed to minimize secondary injury after neurosurgical interventions, traumatic brain injury (TBI), or subarachnoid hemorrhage (SAH). However, no monitor alone can improve patient outcome. Only actions such as decompressive craniectomy for increased intracranial pressure (ICP), based on monitoring data, carry the prospect of improving outcome, and for microdialysis (MD), this aspect requires further studies.
Many neuromonitoring devices attempt to assess changes in cerebral metabolism. This process may be indirectly estimated from global imaging modalities such as xenon computed tomography (CT) and through measuring cerebral oxygen content with jugular venous oxygen saturation (Sjv o 2 ) catheters and cerebral oxygenation (partial pressure of oxygen in brain tissue [Pbt o 2 ]) probes. Direct metabolic substrate measurement in the living human brain currently can only be achieved with cerebral MD catheters or positron emission tomography (PET) scans, which can only be done at great cost and for 1 or 2 time points.
The use of small (approximately 1 mm) semipermiable coaxial and loop membrane tubes within the brain was first described by Bito and colleagues in 1960 and was later refined in 1972 with the advent of the coaxial MD catheter by Delgado and colleagues. In 1986, the first data on neurotransmitter concentrations in the rat brain were reported by Tossman and colleagues.
The first human implantation occurred in 1987 in adipose tissue and was followed in 1990 with the first use in human cerebral tissues. In 1992, the first clinical use of this device was reported, and the creation of commercially available catheters and bedside analysis systems followed shortly. Over time, MD has gained popularity in trauma, stroke, and aneurysmal SAH, and, as more studies verify its usefulness, the indications for use will continue to expand. As of 2012, about 12 centers in North America and about 30 worldwide clinically use brain MD on a regular basis.
MD measurement
The Device
The monitoring device consists of a double-lumen dialysis probe that is implanted within the cerebral tissue ( Fig. 1 ). A physiologic fluid (artificial cerebrospinal fluid [CSF] or normal saline without lactate or glucose) is passed into the probe and over the dialysis membrane using a micropump injector. The concentration gradients between the probe fluid and the surrounding tissue cause various molecules to diffuse across the membrane and into the solution, which is collected and analyzed in sampling vials or passed over a detector system such as a glucose oxidase–based electrochemical sensor. This fluid analysis is not a direct measure of the extracellular concentrations because the concentration in the dialysate depends on the membrane (pore size and surface area), the rate of the fluid flow, the size of the extracellular space, and the individual solutes’ rates of diffusion. It is therefore termed the relative recovery.
There are commercially available 20-kD and 100-kD cutoff membrane probes; however, at the present time, only the 20-kD (CMA70, CMA Microdialysis AB, Solna, Sweden) probe has been approved by the US Food and Drug Administration for human use in the United States and Japan. In Europe, clinical trials with the 100-kD MD probe for the measurement of cerebral intercellular cytokines, neurotrophic factors, and biomarkers are ongoing. A flow rate of 0.3 μL/min is used for 10 mm of membrane length using a 20-kD cutoff membrane. With these settings, the flow recovery rate of CMA70 is approximately 70% for small molecules such as glucose, lactate, pyruvate, glutamate, and glycerol.
Once the device is assembled and secured, the system allows hourly sample collection with bedside, computerized analysis. The device consists of the MD probe, connection tubing, perfusion media, pump, and collection device (see Fig. 1 ). Commercially available assays currently include glucose, lactate, pyruvate, glycerol, and glutamate, in a 12-μL sample aliquot ( Table 1 ). At present, the cost of the setup is approximately $120,000 ($60 per probe and $110,000 for the CMA 600 analyzer and pump). Thus, frequent use lowers the cost substantially per patient.
Parameters | Clinical Significance | Normal Value (Mean ± SD) |
---|---|---|
Glucose | Decrease in hypoxia/ischemia | 1.7 ± 0.9 mmol/L |
Lactate | Increase in hypoxia/ischemia | 2.9 ± 0.9 mmol/L |
Pyruvate | Decrease in hypoxia/ischemia | 166 ± 47 μmol/L |
LPR | Best marker for anaerobic metabolism Increase in hypoxia/ischemia | 23 ± 4 |
Glycerol | Increase with the destruction of cell membrane structure and free radical generation | 82 ± 44 μmol/L |
Glutamate | Increase in hypoxia/ischemia and excitotoxicity | 16 ± 16 μmol/L |
Reimbursement, and Cost Recovery
Several Swedish neurointensive care centers, such as Karolinska, Lund, and Uppsalla have shown that use of MD data can contribute to improved clinical decision making in the most severely injured patients with TBI and SAH. Nursing, critical care, and neurosurgical staff use data from MD to aid decisions, such as vasospasm management, ventilator weaning, choice of oxygen saturation, CSF drainage, and decompressive craniectomy. However, data to justify the cost of MD in terms of improved patient outcome are lacking. In European Union countries, costs have historically been amortized over general patient care and intensive care unit costs, but, in the countries of itemized billing, such as the United States, most centers have funded early MD experience from research grants.
Chen and colleagues recently showed that MD costs can be successfully covered by using inpatient billing codes 99291, and 99292. The procedural code 61105 was introduced to cover twist-drill craniostomy and placement of monitoring catheters with devices such as MD.
Device Implantation
The probe tip is implanted through a twist-drill craniostomy or at the time of surgery. The dura is incised, and the catheter tip is passed to the appropriate depth. This insertion may be done freehand and then tunneled through a skin incision away from the skull defect, or the MD catheter may be a part of a bundled, bolt-based system. The freehand method allows for more precise anatomically selected positioning of the catheter tip but is more labor intensive because the bundle allows for the simultaneous placement of other monitoring devices such as ICP monitors and Pbt o 2 probes.
Because the present system may only sample a small tissue zone, estimated to be about a 10-mm sphere around the catheter tip, several locations are potential targets for device implantation. For TBI, probes placed directly into contusions have been shown to yield severe derangements in metabolism consistent with tissue death and are unresponsive to any manipulations, and therefore this location is not recommended.
Egstrom and colleagues investigated the placement of MD catheters within the penumbra zones as well as within the anatomically normal brain. They found a statistically significant difference in the glucose, lactate, lactate/pyruvate ratio (LPR), glutamate, and glycerol levels between the two catheter locations. Thus, placing the probe in structurally normal tissue may aid in assessing the global cerebral metabolic condition, and placing the probe in pericontusional tissue may provide direct data on the microenvironment of the potentially salvageable tissue. For aneurismal SAH, insertion of the device in the parent artery distribution aids in the detection of vasospasm and may be achieved at surgery for aneurysm clipping.
Standard biomarkers of clinical relevance (with 20-kD MD)
The range of investigational molecule analytes continues to expand, but glucose, lactate, pyruvate, glycerol, and glutamate levels are the current standard for cerebral metabolism available from a 12-μL sample with the CMA analyzer device. These data in combination with other monitoring tools such as ICP monitors, Pbt o 2 probes, Sjv o 2 catheters, electroencephalography, and other modalities are commonly used in the intensive care unit.
Glucose, the primary energy substrate, is initially transported from the extracellular fluid into the cell cytoplasm and, through glycolysis, it is converted to pyruvate. Pyruvate is then aerobically metabolized in the mitochondria through the citric acid (TCA) cycle ( Fig. 2 ). Under normal circumstances, 95% of the cerebral energy requirements come from the aerobic conversion of glucose to water (H 2 O) and carbon dioxide (CO 2 ). Through this process, ATP synthesis is highly efficient and results in the generation of 38 molecules of ATP for each molecule of glucose:
Under hypoxic conditions such as TBI, conversion of glucose can only take place by anaerobic glycolysis because of mitochondrial dysfunction, and only 2 molecules of ATP and 2 molecules of lactate are generated for each molecule of glucose:
With normal aerobic states, pyruvate and the reduced form of nicotinamide adenine dinucleotide (NADH) are taken up by mitochondria, and their oxidation by the TCA cycle and respiratory chain provide most of the ATP production. With the anaerobic state, reoxidation of NADH through the respiratory chain is blocked and must instead occur by the reductive conversion of pyruvate to lactate by lactate dehydrogenase (LDH) (see Fig. 2 ). Thus, an increase of the LPR indicates failure of oxidative phosphorylation and may portend future or ongoing cerebral injury from ischemia or hypoxia.
In general, the LPR normal range averages 23 ± 4 (mean ± standard deviation [SD]), and the mean normal glucose values are 1.7 ± 0.9 mmol/L (see Table 1 ). However, metabolic crisis may occur at a glucose less than 0.8 mmol/L with an LPR greater than 25, and thus several investigators have suggested the MD may indicate brain tissue at risk of delayed damage caused by high ICP or vasospasm.
An additional target molecule, glycerol, is an integral component of cell membranes. The loss of energy caused by ischemia causes an influx of calcium as well as a decomposition of cell membranes caused by free radical activation. When this breakdown occurs, glycerol is released into the interstitial fluid. This mechanism is supported by laboratory studies that show that glycerol generation occurs in proportion to free radical production in the tissues. Thus, glycerol is an important marker of cell membrane damage. The glutamatergic system is also an important mediator for inducing excitotoxic damage in TBI. With brain injury, presynaptic glutamate secretion induces excitotoxicity and an increase in the influx of Ca2+ through N -methyl- d -aspartate (NMDA) receptors. Thus, the glutamate measurements can aid in the evaluation of excitotoxicity after injury.
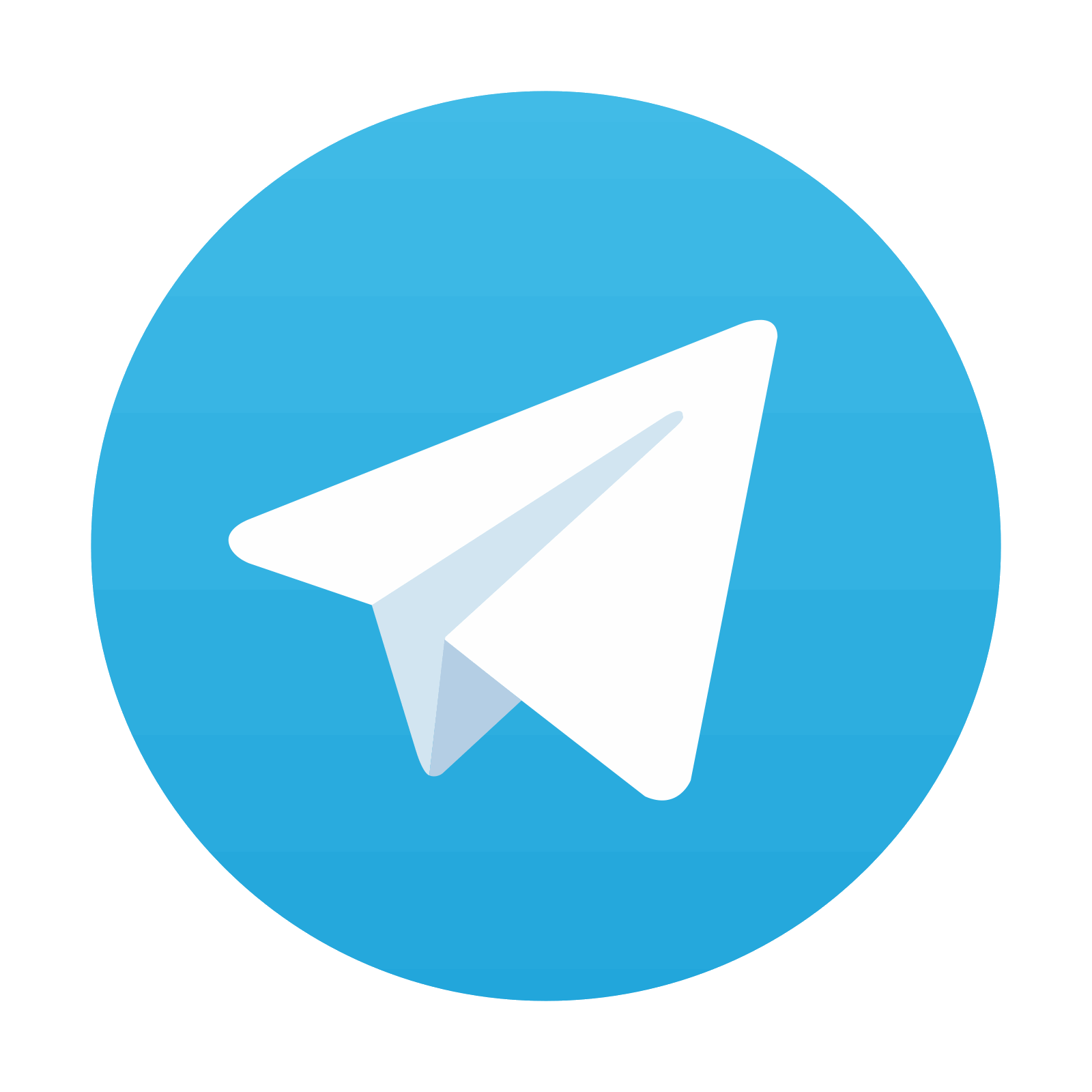
Stay updated, free articles. Join our Telegram channel
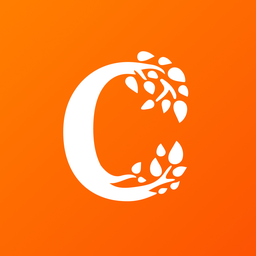
Full access? Get Clinical Tree
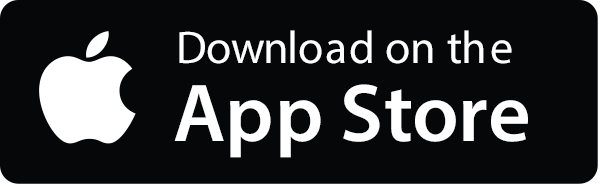
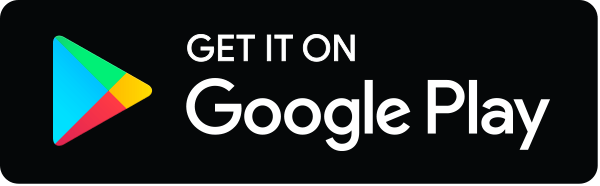