3 Microelectrode Recording Methods Abstract Microelectrode recording (MER) involves the use of high-impedance electrodes that allow precise identification of subcortical structures. MER is routinely employed to reconcile stereotactic targeting based on indirect and direct imaging methods prior to lesion creation or implantation of deep brain stimulator electrodes. Targeting based on anatomical landmarks or direct imaging visualization is prone to error due to individual anatomic variability and imaging distortion. The electrophysiological data generated from MER allows for the correction of stereotactic targeting error and brain shift. Each of the common targets used for neuromodulation for movement disorders demonstrate a unique signature of electrophysiological activity that can allow for detailed mapping with unparalleled precision. MER also has an invaluable role as a research tool, such as for delineating the physiology of deep brain structures in disease states and identifying new targets for deep brain stimulation. Recent advancements in imaging technology and stereotactic technique have allowed for better visualization and targeting of subcortical targets; however, excellent outcomes have been reported with and without MER, and a direct comparison of these techniques has never been performed. The role of MER in neuromodulation surgery will undoubtedly evolve as technologies for intraoperative targeting continue to improve. However, the use of MER continues to be widespread and it remains the most utilized method of target confirmation in movement disorder surgery. Keywords: microelectrode recording, intraoperative mapping, stereotactic targeting, deep brain stimulation, neuromodulation, movement disorders, functional neurosurgery Microelectrode recording (MER) is an important targeting tool for stereotactic surgery and has played a critical role in the development of surgical treatment of movement disorders where clinical efficacy is critically dependent on accurate intraoperative targeting. The modern era of MER can be traced to the early the 1960s when Albe-Fessard and colleagues described the use of low-impedance bipolar electrodes to distinguish between thalamic nuclei and the internal capsule.1,2 This was a significant breakthrough that bridged the recording of single cell action potentials from the laboratory to the clinical setting: not only was MER able to differentiate white matter from gray matter, but it could also discriminate the borders of different subcortical nuclei by resolving each structure’s characteristic neural firing pattern.1 Subsequently, microelectrode technology has been refined to allow precise physiological localization by analyzing the relationship to local field potentials, synchrony with movement or tremor activity, and response to microstimulation.3,4,5,6 Within a few years of its development, MER use for targeting of therapeutic lesions became widespread, but the introduction of levodopa in 1967 led to a transition away from surgery toward medical management that lasted nearly two decades.7,8,9 Nevertheless, experimental and clinical studies using MER continued to facilitate interest in surgery as an important component of movement disorder management10 and in the discovery of new therapeutic targets such as the subthalamic nucleus (STN).11 Moreover, the development of deep brain stimulation (DBS) as a titratable and reversible therapeutic application reinvigorated MER as an adjunct for target localization when placing DBS electrodes.12 Although imaging and intraoperative techniques have improved and now allow for direct anatomic targeting, MER and intraoperative stimulation continue to be widely used in functional neurosurgery. The localization of intracranial targets via radiographic and anatomic means is the critical first step in planning stereotactic procedures, and advances in imaging technologies allow for increasingly greater visualization and identification of therapeutic targets. However, even modern imaging modalities sometimes do not clearly display the target structure due to limitations such as distortion. MER, as an intraoperative adjunct, allows for accurate target localization based on physiological criteria and correction of targeting errors. Furthermore, MER allows precise definition of the ideal physiological target which may differ in some cases from the anatomical target.13 The potential disadvantages of MER include an elevated risk of complications (in particular, hemorrhage) and the increased cost, time, and complexity it adds to the surgical case. The goal of this chapter is to describe the utility of MER for the localization of intracranial targets in stereotactic neurosurgery and outline principles of its use for specific targets. Accurate subcortical target localization can be achieved by careful planning and skilled interpretation of anatomical and/or electrophysiological data. Indirect and direct anatomical visualization via landmarks, stereotactic atlases, and imaging remains the essential first step in planning for movement disorder surgery. However, there are multiple potential sources of error that can be introduced during planning and localization, such as imaging distortion and variability in individual patient anatomy. Furthermore, human and mechanical error must always be considered. MER has the potential to address these sources of error in order to improve target localization. Stereotaxy is prone to small errors, even under optimal conditions, which can add up to several millimeters. These causes of error may include the distortion and spatial inaccuracy of magnetic resonance imaging (MRI), and brain shift due to intraoperative pneumocephalus from cerebrospinal fluid loss.14,15 The passage of a stiff cannula or electrode into the soft brain parenchyma can also cause deflection from the intended target. The use of routine landmarks for the purposes of indirect targeting can be quite variable due to subtle differences in the location of subcortical nuclei.16,17 Critical differences have also been observed in standard stereotactic atlases that are commonly used as overlays during planning.18 MER has the potential to correct for sources of error attributable to spatial inaccuracy, brain shift, or distortion, with real-time feedback and a high degree of accuracy. When targeting is refined using MER data, postoperative imaging sometimes indicates that the corrected electrode position is within the intended target, suggesting that physiological data allowed compensation for a targeting error that would otherwise have been unrecognized. Structure and function do not always correspond, and there may be a difference between physiologic and anatomic targets. MER has the ability to identify physiological structures directly, including structural borders and somatotopic organization. MER can also identify differences in spontaneous firing rates, patterns, and responses to movement between individual nuclei, and it does so with better spatial resolution and accuracy than many MRI sequences. Despite advances in MRI technology, intracranial targets remain challenging to identify. At standard clinical field strength, the globus pallidus is best visualized, the STN somewhat less so, and ventral thalamus least because the radiographic borders of individual thalamic nuclei are indistinct. Precise mapping in three dimensions is possible with MER, including identifying the contours of the nuclei.19 For example, the dorsolateral two-thirds of the STN corresponds to the sensorimotor region and its targeting is optimized with MER and stimulation techniques.20 Precise mapping of nuclear borders can facilitate optimization of the physiological effects of stimulation and prevention of side effects or unintended injury to eloquent structures.21,22 MER and microstimulation are very useful in identifying smaller targets and create smaller lesion tracts compared to macrostimulation, should a new trajectory be required. Furthermore, micro- or macrostimulation alone can produce variable or delayed effects, even at target. Localization via MER is essential when these discrepancies arise, and requires skilled interpretation of neuronal firing patterns.18,23,24 In addition to its clinical use, MER has a valuable role in research. Both historical and contemporary research has relied on MER to help delineate the pathophysiology of movement disorders, the physiology of deep brain structures, and the effect of DBS on downstream neurons. New targets for DBS are being identified with the use of MER, including Parkinson’s disease (PD)-associated freezing of gait25,26 and epilepsy.27 The methodology for target localization and use of MER varies widely between neurosurgical centers; however, the general setup and technical aspects of MER insertion and recording are standard. In general, the essential components include the microelectrode or semi-microelectrode, audio/video monitors, and an amplifier/oscilloscope. A motorized microdriver for electrode advancement is often used that must be compatible with the available stereotactic targeting system. Microdriver systems may be hydraulic or motorized, with the latter allowing electrode advancement via an electronic control. Intraoperative stimulation can be performed using a current isolation stimulator, and stimulation is either passed through the high-impedance recording contact (microstimulation) or referential contact (macrostimulation). The microelectrode usually consists of a tungsten or platinum-iridium bipolar electrode with a glass-insulated tapered tip having a diameter of 5 μm (or less) and a length of 10 to 15 μm for recording. Microelectrodes have high impedances (greater than 500 kΩ) that allow for single unit isolation in regions with a high density of neurons, and thus better target differentiation during recording.28,29 Semi-microelectrodes have a larger tip diameter (25 μm or larger) with lower impedance resulting in greater sensitivity to neural activity at the expense of inability to discriminate single-cell recordings, thus providing less specific information.30 The physical properties of the electrodes ensure durability to microstimulation, although the passage of current degrades the glass insulation and lowers the impedance, resulting in decreased noise but increased difficulty in isolation of single units. The microelectrode is inserted within a protective stainless-steel cannula that allows for stability and retraction of the electrode tip during advancement through brain parenchyma. The MER apparatus is first positioned at a predetermined distance above the intracranial target (typically 15–25 mm) and the electrode is slowly advanced to target and a short distance beyond as recording is performed. During microelectrode advancement, the signal is filtered and amplified via isolated preamplifiers and processed through low- and high-pass filters to eliminate excess noise. An oscilloscope displays this filtered signal that is often enhanced through digital processing. Sound is also transmitted through speakers, and the signal is heard as multiple discharges. Individual neuronal firing activity can be isolated by using window discriminators. There are many different philosophies about how MER should be used and how much electrophysiological detail is necessary. For example, electrode passage along a single trajectory can verify the presence of the target structure and define its depth along that specific trajectory. An alternative option is to employ multiple parallel passes to create detailed three-dimensional maps that define the borders of multiple subcortical structures. Multiple electrodes can be simultaneously inserted and recorded from during a single pass using a multichannel microarray. One such example, the “Ben gun,” has one central channel and four side channels positioned 2 mm from the central channel. This device allows the advancement of up to five parallel microelectrodes simultaneously.31 This technique allows the user to directly compare recordings between electrodes, and a theoretical volume of tissue can be delineated given the fixed distance between the electrodes.32 MER can be used alone or in conjunction with micro- or macrostimulation delivered via an isolated current stimulator. Recordings from MER are accurate to about 0.1 mm, whereas stimulation current spreads over a much wider area of up to a few millimeters. Thus, mapping by stimulation is somewhat less precise. Stimulation is performed using monopolar or bipolar biphasic charge-balanced square wave pulse trains of 0.06 to 0.3 milliseconds at 130 to 300 Hz. Depending on the position of the electrode, a variety of sensory, pyramidal, or extrapyramidal manifestations can assist in physiological mapping. It is imperative to have a trained practitioner for interpretation of these manifestations, as the physiological manifestations of stimulation can vary on the basis of location. Although documenting changes in tremor may be straightforward to quantify, tremor arrest may also be seen in areas outside of target, such as the corticospinal tract and zona incerta. Stimulation also tends to be accurate to approximately 5 mm of targeted tissue,32 and this can produce false negatives. The thalamus was an important early stereotactic target in the treatment of PD as thalamotomy provides significant relief of tremor, and the ventral thalamic nuclei are commonly targeted with DBS to treat movement disorders. These nuclei include: the ventral oral (Vo) or pallidal relay nucleus targeted for parkinsonism or dystonia; the ventral intermediate (Vim) or cerebellar relay nucleus targeted for tremor; and the ventral caudal (Vc) or somatic sensory nucleus targeted to aid in localization of the Vim and also to treat certain neuropathic pain syndromes.33,34 These targets are not clearly visualized on MRI; thus, radiological determination of the anterior and posterior commissures (AC-PC line) is first performed which allows for “indirect” targeting based on standard coordinates. Targeting may be aided by using preprogrammed atlas maps which approximate the location of nuclei based on the AC-PC line or other surrounding structures. Physiological localization can then be performed with recording and/or stimulation with a micro- or macroelectrode to identify the thalamic nuclei based on their unique electrophysiological responses. The Vim is organized somatotopically, with face medial and leg lateral. The Vim also contains “kinesthetic cells” which respond to passive joint movements and demonstrate firing synchronous with tremor activity.35,36,37 These kinesthetic cells demonstrate variable firing rates depending on the disease pathology: tremor cells fire in rhythmic bursts with clinical tremor activity, and in essential tremor these fire at a higher frequency compared with PD or pain.34,38 Tremor cells have been theorized to cluster approximately 2 mm anterior to the anterior border of Vc, and 3 mm above the AC-PC line.39 The anterior Vo (Voa) nucleus has been proposed as a superior target for rigidity control, whereas the posterior Vo (Vop) is preferred for tremor.40,41 MER of the Vop has also demonstrated rhythmic bursting activity at tremor frequencies. Vc is sometimes chosen for initial localization with MER, as it is the most easily identifiable of all the ventral thalamic nuclei, and the final electrode trajectory can then be fine-tuned based on the electrophysiological findings. Due to the frontal angle of entry, the electrode is likely to first enter the Vop, then Vim, before entering Vc. Neuronal activity within the Vop and Voa correlates with movement in response to commands, the active phase of movement, and a state of maximal muscle contraction.36,42,43 As such, spontaneous firing activity is lower compared with other targets. So-called voluntary cells produce the largest proportional change in firing rates in response to active movement, and tremor cells may be encountered that fire in synchrony with the patient’s tremor. The execution of particular movements often preferentially relates to a specific firing pattern, and the somatotopy is parallel to the cutaneous core of Vc.42 Vim and Vop are readily identified by the response to stimulation of deep structures, such as tendons, or movement of joints and phasic tremor frequency. Certain cells also respond to both somatosensory stimulation and active movement. In addition, Vop is identifiable by EEG spindle activity with a 7 to 10-Hz rhythm, and increases and decreases in amplitude.34 Posterior to Voa and Vop and anterior to the cutaneous core in the anterior dorsal cap of Vc is the Vim which contains “kinesthetic cells” that respond primarily to passive joint movement, deep pressure, and squeezing of muscles and tendons, but not manipulation of skin deformed by these movements. Although there is some activation with voluntary movement, the amplitude is often equal or less than that produced by passive movement. Kinesthetic cells may fire concurrently with tremor if the receptive fields overlap.44 Vim and Vc share a similar somatotopy, with deep sensory cells of the wrist anterior to the cutaneous representation of digits.45,46 The border between Vim and Vc can be difficult to identify, as macrostimulation produces paresthesias within similar parts of the body. However, threshold for activation tends to be higher in Vim.
3.1 Introduction
3.2 The Rationale for Mapping
3.3 Microelectrode Technology and Technique
3.3.1 Ventral Thalamus
Microelectrode mapping of Voa/Vop, Vim, and Vc
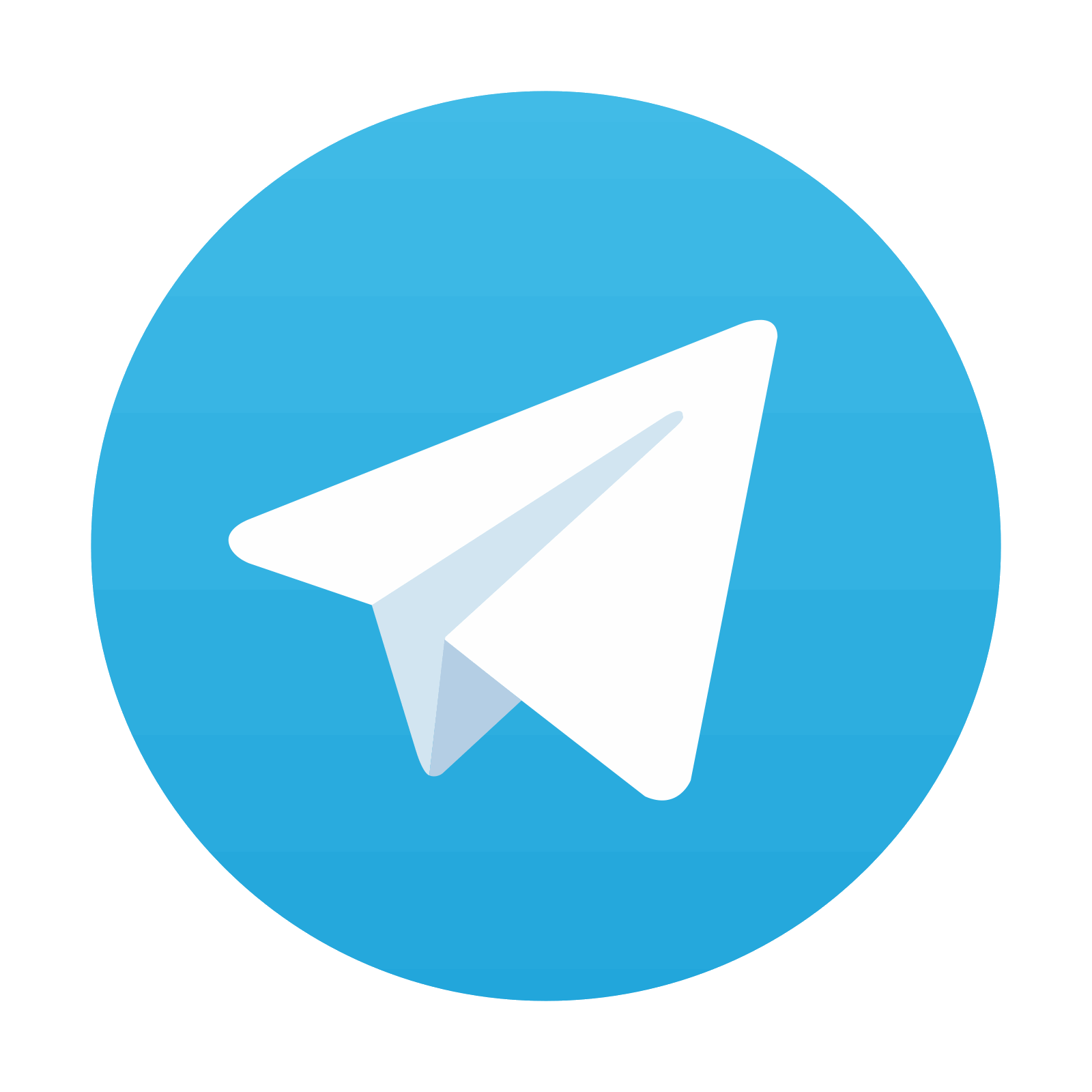
Stay updated, free articles. Join our Telegram channel
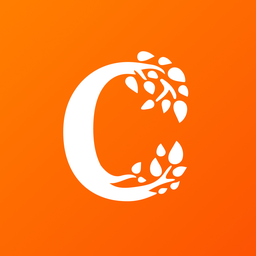
Full access? Get Clinical Tree
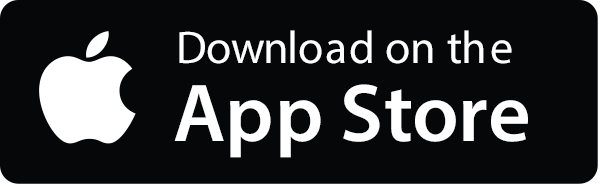
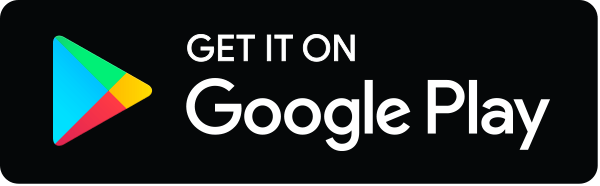