Fig. 17.1
miRNA biogenesis. miRNAs are encoded in the genome (inter or intragenis) and transcribed by RNA polymerase II to generate primary microRNA (pri-miRNA). These pri-miRNAs are taken up by Drosha/DGCR8, which catalyzes the formation of precursor miRNA (pre-miRNA). pre-miRNA is then exported to cytoplasm by Exportin5 in conjunction with Ras-related nuclear protein, RanGTP. In the cytoplasm, pre-miRNA is cleaved into miRNA:miRNA* duplex by Dicer/Tar RNA-binding protein (TRBP) or PKR activating protein (PACT). One of these miRNA/miRNA* duplexes is discarded and the other one is loaded onto an Argonaute homolog protein (Ago, isoforms of eIF2c) to generate the effector complex, known as RNA-induced silencing complex (RISC). The RISC complex directs miRNA to specific “short-seed” sequences located predominantly within the 3-UTR region of the target mRNA. This leads to degradation or translational inhibition
17.2 Possible Mechanisms of miRNA Regulation of Target Genes
RISC is critical in recognizing specific “short-seed” sequences located predominantly within the 3′ untranslated region (3′UTR) of target mRNAs. The binding of RISC to these short-seed sequences leads to interference in the translation of mRNA. miRNA-mediated translational inhibition also depends upon the 5′cap region of the target mRNA. Ago proteins can stimulate miRNA-dependent inhibition of translation by competing with elongation factor eIF4E for the 5′cap binding site, thus preventing circularization of mRNA and lowering initiation efficiency (Mathonnet et al. 2007). Because the RISC/miRNA complex recognizes target mRNA on a seed region containing 2–8 nucleotides at the 5′end of mRNA, it provides a mechanism by which one miRNA can target several mRNAs (Brodersen and Voinnet 2009). RISC can also associate with 60S ribosome and eIF6 (Chendrimada et al. 2007). eIF6 regulates the formation of the transnationally active 80S subunit. By regulating eIF6, miRNAs can modify polysome formation and expose target mRNAs for degradation (Chendrimada et al. 2007). In certain cases, miRNAs may actually enhance, rather than inhibit, translation (Vasudevan et al. 2007). miRNA-mediated regulation of mRNA stability is another mechanism by which miRNAs suppress expression of specific mRNA (Wu et al. 2006).
Besides regulating translational process, it has been shown that miRNA can also regulate gene transcription by targeting transcription factors. In this case, levels of transcription factors are downregulated by miRNAs, which in turn cause less expression of mRNA, leading to reduced protein synthesis (Kosik 2006; Michalak 2006). miRNA biogenesis can also be regulated at the epigenetic level (Tardito et al. 2013). For example, inhibitors of DNA methylation and histone deacetylases can affect expression of several miRNAs (Chuang and Jones 2007). On the other hand, a subset of miRNAs can control the expression of epigenetic regulators, such as DNA methyltransferases, histone deacetylases, and polycomb group genes, leading to transcriptional activation of protein coding gene sequences (Sato et al. 2011).
17.3 miRNAs and Synaptic Plasticity
17.3.1 Synaptic Plasticity and Regulation of BDNF and CREB by miRNAs
miRNAs play a critical role in regulating synaptic plasticity, a putative core feature of depression pathophysiology. Knocking down the RNase III enzyme Dicer leads to reduction in neuronal size, and branching, as well as aberrant axonal pathfinding (Davis et al. 2008; De Pietri Tonelli et al. 2008; Schaefer et al. 2007). DGCR8 knockout mice show a loss of synaptic connectivity, reduced number, and size of the dendritic spines (Olde Loohuis et al. 2012; Stark et al. 2008), and interestingly, impaired spatial working memory-dependent tasks (Stark et al. 2008). Such memory deficits are often associated with depressive symptoms. FMRP, which regulates protein synthesis in dendritic spines after binding with specific sites within the 3′UTR of certain mRNAs in concert with RISC components Ago1and Dicer (Jin et al. 2004; Qurashi et al. 2007), is also associated with learning, memory, and associated long-term potentiation (LTP). For example, the RISC protein armitage is essential for LTP and synaptic protein synthesis and is cleaved during the learning process (Ashraf et al. 2006).
FMRP is associated with miR-132 and miR-125b in the brain. miR-132 overexpresion increases dendritic protrusion as well as branching (Wayman et al. 2008), whereas miR-125b targets NR2A mRNA and regulates synaptic plasticity in a negative fashion (Edbauer et al. 2010). Similar negative regulation of the size of dendritic spines in rat hippocampal neurons has been shown to be associated with miR-138 as well as miR-134. miR-138 controls the expression of acyl protein thioesterase 1 (APT1), an enzyme regulating the palmitoylation status of proteins that are known to function at the synapse (Siegel et al. 2009). miR-134 additionally inhibits translation of Lim-domain-containing protein kinase 1 (Limk1) (Schratt et al. 2006), a protein that regulates dendritic spine growth (Meng et al. 2004). Exposure to BDNF relieves the suppression of Limk1 translation caused by miR-134. miR-134 can also promote denditogenesis by inhibiting translational repressor Pumilio2 (Fiore et al. 2009). This is consistent with the finding that BDNF has been shown to be lower in brains of depressed suicide subjects (Dwivedi et al. 2003a, 2009a).
Several studies have demonstrated that CREB, a key transcription factor regulating synaptic plasticity and whose expression is lower in depressed and suicidal brain (Dwivedi et al. 2003b) is one of the major targets of several miRNAs (Vo et al. 2005). Conversely, many miRNAs have binding sites in their promoter regions for CREB (Wu and Xie 2006). Expression of miR-132, which enhances neurite outgrowth, dendritic morphogenesis, and spine formation (Vo et al. 2005; Impey et al. 2010), is induced by BDNF via CREB. Another miRNA, miR-124, which plays a significant role in maintaining neuronal cell identity, is a major target of CREB. Interestingly, miR-124 is rapidly and robustly regulated by 5-HT, which selectively affects mature miR-124 levels, without affecting its precursor, suggesting that the miR-124 level may be regulated during Dicer processing or RISC incorporation and stabilization by Ago (Rajasethupathy et al. 2009). miR-124 responds to serotonin by de-repressing CREB and thereby enhances serotonin-dependent long-term facilitation (Rajasethupathy et al. 2009). More recently, it has been shown that expression of SIRT1 gene, which modulates synaptic plasticity and memory formation, is altered via posttranscriptional regulation of CREB by miR-134 (Gao et al. 2010). Conversely, SIRT1 inhibits the expression of miR-134 via a repressor complex containing the transcription factor YY1. Unchecked miR-134 expression after SIRT1 deficiency may result into the reduced expression of CREB and BDNF, whereas knocking down miR-134 rescues LTP and memory impairment caused by SIRT1 deficiency (Gao et al. 2010).
CREB- and activity-regulated miR132 is necessary for hippocampal spine formation (Impey et al. 2010). Expression of the miR-132 target, p250GAP, is inversely correlated with miR-132 and spinogenesis. Knockdown of p250GAP increases spine formation while introduction of a p250GAP mutant unresponsive to miR132 attenuates this activity. Inhibition of miR132 decreases both mEPSC frequency and the number of GluR1-positive spines, while knockdown of p250GAP has the opposite effect. Additionally, miR132/p250GAP circuit regulates Rac1 activity and spine formation by modulating synapse-specific Kalirin7-Rac1 signaling. These studies suggest that neuronal activity regulates spine formation, in part, by increasing miR132 transcription, which in turn activates a Rac1-Pak actin remodeling pathway (Impey et al. 2010). Behaviorally, it has been shown that overexpression of miR-132 in the rat perirhinal cortex impairs short-term recognition memory, which is associated with a reduction in both long-term depression and long-term potentiation (Scott et al. 2012).
17.3.2 Synaptic miRNAs: Direct Regulator of Plasticity
Synaptic efficacy is critically dependent upon regulation of specific protein synthesis near or within synapse (Weiler et al. 1997; Todd et al. 2003; Sutton and Schuman 2005). miRNAs provide fascinating mechanisms of regulating synaptic efficacy and plasticity by modifying translational control of protein synthesis locally at the synapse. Synaptic enrichment of miRNAs in mouse forebrain synaptosomes has extensively been characterized (Lugli et al. 2005; Smalheiser 2008). These studies show that a significant subset of miRNAs is highly enriched in synaptic fractions relative to total homogenate. These synaptic-enriched miRNAs were biologically distinct from synaptic-depleted miRNAs, both in their expression patterns (synaptic-enriched miRNAs are expressed primarily in pyramidal neurons, whereas synaptic-depleted miRNAs tend to have widespread and abundant tissue expression) and in their evolutionary histories (synaptic-enriched miRNAs tend to be evolutionarily new, often mammalian-specific, whereas the synaptic-depleted miRNAs tend to be highly conserved across vertebrates). Interestingly, the synaptic enrichment was not simply related to specificity of miRNA expression within neurons, rather, they arise from precursors that are expressed in the synaptic fractions and associated tightly with post-synaptic density (PSD) (Smalheiser 2008). Furthermore, synaptic enrichment of miRNAs has been shown to be related to structural features of their precursors, suggesting a basis by which pre- or pri-miRNA may be selectively and stably transported to dendrites (Budhu and Wang 2010). Because both dicer and pre-RNAs are expressed in synaptic fractions and are strongly associated with PSDs, it suggests that at least a portion of the mature miRNAs is locally processed near synapses. Because of the critical feature of miRNAs to regulate gene circuitry locally at the synapse in an activity-dependent fashion, one can modulate synaptic efficacy by modulating miRNA functions at the synapse and consequently synaptic plasticity. This provides unique opportunity at therapeutic level, where regulation of miRNA can be used to control plasticity at the synapse.
17.4 miRNAs and Stress
Glucocorticoids regulate the hypothalamic-pituitary adrenal axis through a negative feedback mechanism while binding to soluble glucocorticoid receptors (GRs) in the pituitary and the hypothalamus and inhibit the release of corticotropin-releasing factor and adrenocorticotropic hormone. Expression of GRs are downregulated in depressed individuals (Pariante and Miller 2001). GR protein is under constant regulation by miRNAs (Vreugdenhil et al. 2009); specifically, miR-124a and miR-18a bind to 3′UTR of GR gene and downregulate its expression (Vreugdenhil et al. 2009). Overexpression of miR-18a attenuates glucocorticoid-induced leucine zipper, a gene induced by stress-like levels of glucocorticoid. Interestingly, Uchida et al. (2008) found that miR-18a-mediated downregulation of GR translation is important in susceptibility to stress. Turner et al. (2010) recently predicted several possible miRNA binding sites within the GR first exon, suggesting regulation of GR genes by miRNAs.
Several types of stressors have been utilized to examine how miRNAs respond to these stressors. Using unpredictable chronic mild stress combined with separation, Cao et al. (2007) found changes in 13 specific miRNAs in rat hippocampus. These include: downregulating miRNAs miR298, miR-130b, miR-135a, miR-323, miR-503, miR-15b, miR-532, and miR-125a and upregulating miRNAs miR7a, miR-212, miR-124, miR-139, and miR-182. Among these, miR-125a and miR-182 recovered to normal after intervention with antidepressant medication.
Acute and chronic restrained stress cause differential changes in expression of miRNAs in a brain region-specific manner (Rinaldi et al. 2010). For example, acute stress induces a transient increase in the expression of miR-9, miR-9*, miR-26b, miR-29b, miR-30b, miR-30c, miR-30e, miR-125a, miR-126-3p, miR-129-3p, miR-207, miR-212, miR-351, miR-423, miR-487b, miR-494, miR-690, miR-691, miR-709, miR-711, and Let-7 a-e in the frontal cortex but not in hippocampus. Some of these miRNAs (let-7a, miR-9, miR-26a/b, miR-30b/c, and miR-125a) show increase in their expression 5 days after acute stress. Interestingly, their expression levels are not altered after repeated restraint. These results suggest that acute stress modulates miRNA expression rapidly to external stimuli, which could be due to altered synaptic efficacy through regulation of localized mRNA translation.
Using chronic immobilization stress paradigms, Meerson et al. (2010) also found that the expression of several miRNAs was differentially altered in the central amygdala and the CA1 region of the hippocampus of rats during acute and chronic stress; chronic stress causing much larger changes than acute stress. Some of the miRNAs that were altered during acute and chronic stress include: miR-134, miR-183, miR-132, Let-7a-1, miR-9-1, and miR-124a-1. Interestingly, except for miR-Let-7a-1, the expression of stress-responsive miRNAs were different in the two analyzed brain regions. In the CA1 region, miR-376b and miR-208 increased whereas miR-9-1 decreased under both acute and chronic stress conditions. Stress-responsive miR-134 and miR-183 target many splicing factors, such as SC35, SRP46, and SFRS11. SC35 promotes the alternative splicing of acetylcholinesterase from the synapse-associated isoform AChE-S to soluble AChE-R protein and the expression of SC35 is increased during stress. Thus, by regulating splicing factors and their targets, miR-183 and miR-134 may modify both alternative splicing and cholinergic neurotransmission in the stressed brain. In addition, one of the targets of miR-183 is profilin 2 mRNA, which regulates dendritic spine morphology in neurons. Interestingly, neurotransmitter homeostasis and behavior are severely affected in profilin 2 knockdown mice (Witke 2004) and PFN2 expression is increased in lymphoblastoid cell lines of monozygotic twin pairs discordant for bipolar disorder (Matigian et al. 2007).
17.5 miRNAs in Animal Models Depression-Like Behaviors
An individual’s ability to cope with stress is critical in the development of depression. miRNA expression was recently examined in frontal cortex of rats which developed behavior (learned helpless [LH]) that resembles stress-induced depression and in at those which did not develop depression-like symptoms (non-learned helpless [NLH]) despite similar exposure to inescapable shock (Smalheiser et al. 2011). NLH rats showed a robust adaptive miRNA response to inescapable shocks whereas LH rats showed a markedly blunted miRNA response. One set of miRNAs showed large, significant, and consistent alterations in NLH rats, consisting of miR-96, miR-141, miR-182, miR-183, miR-183*, miR-198, miR-200a, miR-200a*, miR-200b, miR-200b*, miR-200c, and miR-429. All were downregulated in NLH rats relative to tested controls (no-shock group), and all showed a blunted response in LH rats. These miRNAs were encoded at a few shared polycistronic loci, suggesting that their downregulation was coordinately controlled at the level of transcription. Most of these miRNAs have previously been shown to be enriched in synaptic fractions (Lugli et al. 2008). Moreover, almost all of these miRNAs share 5′-seed motifs with other members of the same set, suggesting that they will hit similar or overlapping sets of target mRNAs. Interestingly, half of this set are predicted to hit CREB1 as a target, and binding sites for CREB lie upstream of miR-96, miR-182, miR-183, miR-200a, miR-200b, miR-200c, miR-220a*, and miR-200b*. This suggests that a similar feedback loop arrangement may also exist for CREB as well, similar to what has been described for other CREB-stimulated miRNAs and target genes (Wu and Xie 2006). Because these miRNAs are downregulated in NLH rats, but not LH rats, this can be interpreted as a homeostatic response intended to minimize repressive effects on CREB1. In addition, a large core coexpression module was identified (Smalheiser et al. 2011), consisting of miRNAs that are strongly correlated with each other across individuals of the LH group, but not either the NLH or tested control group. The presence of such a module implies that the normal homeostatic miRNA response to repeated inescapable shock is not merely absent or blunted in LH rats; rather, gene expression networks are actively reorganized in LH rats, which may support their distinctive persistent phenotype.
In further support of the suggestion that miRNAs can affect depressive-like behavior, Enoxacin, a fluoroquinolone used clinically as an antibacterial compound that also enhances the production of miRNAs in vitro and in peripheral tissues in vivo, can affect depressive behavior. Treatment of rats with 10 or 25 mg/kg enoxacin for 1 week increased the expression of miRNAs in frontal cortex and decreased the proportion of rats exhibiting learned helpless behavior following inescapable shock, suggesting that enoxacin may ameliorate depressive behavior, possibly due to upregulation of miRNAs (Smalheiser et al. 2014).
17.6 miRNAs in Depression and Suicide: Human Postmortem Brain Studies
The role of miRNAs in depression and suicide is still in infancy and so far, there have been only a handful of studies investigating the role of miRNAs in depression and suicide. miRNA expression in prefrontal cortex of depressed subjects who died by suicide was recently characterized (Smalheiser et al. 2012), finding that 21 miRNAs were significantly downregulated in the MDD group. 24 additional miRNAs were downregulated by 30 %. Although not statistically significant, the findings suggest a global downregulation of miRNAs levels in the MDD group. When analyzed individually, almost half of the downregulated miRNAs were encoded at chromosomal loci near another miRNA and are possibly transcribed by the same pri-miRNA gene transcripts (miR-142-5p and 142-3p; miR-494, 376a*, 496, and 369-3p; miR-23b, 27b, and 24-1*; miR-34b* and 34c; miR-17* and 20a). In addition, three pairs of miRNAs were encoded at distances greater than 100 kb but still found to lie within the same chromosomal region (miR-424 and 20b at Xq26.2-3, 377 kb apart; miR-142 and 301a at 17q22, 820 kb apart; miR-324-5p and 497 at 17p13.1, 205 kb apart). This suggests that at least some of the downregulated miRNA expression is due to decreased transcription. Many of the downregulated miRNAs also shared 5′-seed sequences that are involved in target recognition. For example, identical seed sequences are shared by (a) miR-20a and 20b; (b) miR-301a and 130a; and (c) miR-424 and 497. As well, a 6-mer nucleotide motif is shared by miR-34a, 34b*, and 34c, and strikingly, a 5-mer motif (AGUGC) within the 5′-seed is shared by five of the affected miRNAs (miR-148b, 301a, 130a, 20a, and 20b) that is predicted to bind Alu sequences within the 3′-UTR region of target mRNAs. This suggests that the downregulated miRNAs should exhibit extensive overlap among their mRNA targets.
When pair-wise correlations were made (a complementary method of analyzing the miRNA expression data is to identify pairs of miRNAs that are co-regulated in their expression, up or down, across individuals within a single group), a set of 29 miRNAs were identified, none of which were pair-wise correlated in the normal control group, but which formed a very extensive interconnected network in the depressed group. Several of the miRNAs (let-7b, miR-132, 181b, 338-3p, 486-5p, and 650) were “hubs” correlated with four to nine other miRNAs in the network. Target analysis revealed that many of the targets are transcription factors, and nuclear, transmembrane, and signaling proteins. Intriguingly, four different downregulated miRNAs target VEGFA (miR-20b, 20a, 34a, and 34b*), a molecule implicated in depression in both humans and in animal models. Other validated targets include BCL2 (miR-34a), DNMT3B (miR-148b), and MYCN (miR-101, 34a). Among predicted targets, estrogen receptor alpha, ESR1, was predicted to be targeted by three different downregulated miRNAs (miR-148b, 301a, and 496). Others targeted by three or more affected miRNAs include ubiquitin ligases (UBE2D1 and UBE2 W), signal transduction mediators (CAMK2G, AKAP1), the splicing factor NOVA1 that regulates brain-specific alternative splicing; the GABA-A receptor subunit GABRA4; calcium channel CACNA1C; and brain-active transcription factors including SMAD5, MITF, BACH2, MYCN, and ARID4A. Several of these predicted targets interact with validated targets; for example, ARIA4A binds E2F1; SMAD5 binds RUNX1; and estradiol treatment decreases E2F1 levels in prefrontal cortex (Wang et al. 2004). BACH2 transcription factor binding sites have been identified upstream of many brain-expressed miRNAs (Wu and Xie 2006). Retinoblastoma binding protein 1 (ARIA4A) is of interest because it recruits histone deacetylases and regulates gene expression via chromatin-based silencing.
Selected target proteins such as DMNT3b, VEGFA, and BCL2 were studied by examining their expression in depressed suicide brain. DMNT3b was strongly upregulated in the depressed suicide group, whereas BCL2 was downregulated. Several miRNAs that were coregulated with their targets showed a strong positive correlation with DMNT3b and BCL2. A variety of factors such as transcription factor activity and turnover rate, as well as possible regulatory effects of other miRNAs may also be responsible for changes in mean expression levels of these target proteins. In addition, DNMT3b levels showed an extremely strong positive correlation with miR-148b across subjects (r = 0.91 in controls, r = 0.94 in the depressed suicide group). Similarly, BCL2 was strongly and positively correlated with miR-34a (r = 0.92 in healthy controls, r = 0.82 in the depressed suicide group). The correlation of miR-34a was positive in healthy controls, but inverse in the depressed suicide group, presumably reflecting a reorganization of miRNA-target networks (Smalheiser et al. 2012).
Previous studies indicate that TrkB-T1, a BDNF receptor lacking a tyrosine kinase domain that is highly expressed in astrocytes and regulates BDNF-evoked calcium transients, is downregulated in frontal cortex of suicide subjects (Ernst et al. 2009, 2011). In a recent study, Maussion et al. (2012) examined whether this TrkB-T1 gene is regulated by miRNAs. The investigators found that Hsa-miR-185* and Hsa-miR-491-3p were upregulated in suicide completers with low expression of TrkB.T1 (P(nominal): 9.10(−5) and 1.8.10(−4), respectively; FDR-corrected p = 0.031). Bioinformatic analyses revealed five putative binding sites for the DiGeorge syndrome-linked miRNA Hsa-miR-185*in the 3′UTR of TrkB-T1, but none for Hsa-miR-491-3P. The increase of Hsa-miR-185* in frontal cortex of suicide completers was validated then confirmed in a larger, randomly selected group of suicide completers, where an inverse correlation between Hsa-miR-185* and TrkB-T1 expression was observed (R = −0.439; p = 0.001). Silencing and overexpression studies performed in human cell lines confirmed the inverse relationship between hsa-mir-185* and trkB-T1 expression. Luciferase assays demonstrated that Hsa-miR-185* binds to sequences in the 3′UTR of TrkB-T1. These results suggest that an increase of Hsa-miR-185* expression levels regulates, at least in part, the TrkB-T1 decrease observed in the frontal cortex of suicide completers and further implicate the 22q11 region in psychopathology.
Alterations in metabolic enzymes of the polyamine system have been reported to play a role in predisposition to suicidal behavior (Fiori et al. 2011). Recently, Lopez et al. (2014) examined whether dysregulation of polyamine genes in depressed suicide completers could be influenced by miRNA post-transcriptional regulation. These investigators identified several miRNAs that target the 3′UTR of polyamine genes SAT1 and SMOX. When the expression of 10 miRNAs in the prefrontal cortex of suicide completers and controls using qRT-PCR were profiled, they found that several miRNAs showed significant up-regulation in the prefrontal cortex of suicide completers compared to psychiatrically-healthy controls (miR-124, miR-139-5p, miR-195, miR-198, miR-320c, miR-33b, miR-34a, miR-34c-5p, miR-497, miR-873). However, they found that only miR-139-5p, miR-320c were inversely correlated with polyamine gene SAT1 whereas miR34c-5p and miR-320c were inversely correlated with polyamine gene SMOX. These results suggest a relationship between miRNAs and polyamine gene expression in the suicide brain, and postulate a mechanism for SAT1 and SMOX down-regulation by post-transcriptional activity of miRNAs.
17.7 miRNAs in Clinical Diagnosis of Depression and Suicidal Behavior: Role of Circulating miRNAs
miRNA can be detected in circulating biological fluids such as serum, plasma, urine, saliva, and CSF (Cogswell et al. 2008; Hanke et al. 2010; Weber et al. 2010). Under healthy conditions, these miRNAs are stably expressed in blood cells; however, under pathological conditions, the profile of miRNAs changes significantly, suggesting that peripheral miRNAs can possibly be used as a reliable biomarker under disease conditions. Recently, blood cell miRNAs have been shown to be extremely useful for detecting and following the course of human cancer, myocardial infarction, and neurodegenerative conditions, including Alzheimer’s disease, Parkinson disease, Huntington disease, and prion disease (Chen et al. 2008; Fichtlscherer et al. 2010; Ma et al. 2013; Dorval et al. 2013; Jin et al. 2013).
Circulating miRNAs arise from heterogeneous sources and are expressed within different compartments of blood. For neuropsychiatric perspective, it is imperative to characterize miRNAs in blood cells that are brain derived. In this respect, it has been shown that miRNAs can be released actively or passively from neurons into the circulating blood (Fichtlscherer et al. 2010). These miRNAs are enclosed in exosomes or microvesicles and are protected by RNA-binding proteins.
The use of miRNAs as peripheral biomarker in depression is gaining momentum. Belzeaux et al. (2012) examined the miRNA expression profile in peripheral blood mononuclear cells (PBMCs) collected from 16 severely depressed patients and 13 matched controls at baseline, 2 and 8 weeks after treatment. miRNAs that showed changes between depressed and controls at baseline included: has-miR-107, miR-133a, miR-148a, miR-200c, miR-381, miR-425-3p, miR-494, miR-517b, miR-579, miR-589, miR-636, miR-652, miR-941, and miR-1243. Only 2 miRNAs showed stable overexpression in depressed patients during the 8-week follow-up compared with controls (miR-941 and miR-589). They also identified miRNAs exhibiting significant variations of expression among patients with clinical improvement (7 upregulated and 1 downregulated). Fourteen dysregulated miRNAs had putative mRNA targets that were differentially expressed in depressed subjects, suggesting a common RNA regulatory network functions in depression. These results also suggest the potential utility of miRNA signatures as markers of depressive episode evolution.
Bocchio-Chiavetto et al. (2013) conducted a whole-miRNome quantitative analysis in the blood of 10 MDD subjects after 12 weeks of treatment with escitalopram. They found that 30 miRNAs were differentially expressed after the escitalopram treatment: 28 miRNAs were upregulated, and 2 miRNAs were downregulated. Thirteen of them (let-7d, let-7e, miR-26a, miR-26b, miR-34c-5p, miR-103, miR-128, miR-132, miR-183, miR-192, miR-335, miR-494, and miR-22) play a role in the neural plasticity and stress response and in the pathogenetic mechanisms of several neuropsychiatric diseases. miR-132 exerts critical functions in the biological circuits implicated in neurogenesis and synaptic plasticity, stimulating axonal and dendritic outgrowth in different brain areas (Mellios et al. 2011). This miRNA, together with miR-26a, miR-26b, and miR-183, widely contributes to the action of the neurotrophin BDNF in the brain (Wayman et al. 2008; Caputo et al. 2011; Kawashima et al. 2010). miR-132, miR-26a, miR-26b, miR-183, let-7d, let-7e, miR-26b, miR-103, miR-128, miR-494, and miR-22 have been reported to play a role in the pathogenesis of psychiatric disorders and in the mechanism of action of antipsychotic drugs and mood stabilizers. Moreover, postmortem studies in the brains of bipolar disorder patients show increased levels of miR-22* in the prefrontal cortex (Kim et al. 2010). On the other hand, miR-494 and miR-335 are downregulated in the prefrontal cortex of depressed suicide patients (Smalheiser et al. 2012). The target genes of these altered miRNAs include: BDNF, GR, NR3C1, and the nitric oxide synthase NOS1, growth factors (IGF1, FGF1, FGFR1, VEGFa, and GDNF), calcium channels (CACN41C, CACNB4, SLC6A12, and SLC8A3), and neurotransmitter receptors (GABRA4 and 5-HT4), some of which have been implicated depression and in the mechanism of action of antidepressants. These studies suggest that miRNAs can not only be used to diagnose but can also be used for treatment response.
More recently, He et al. (2012) studied an association between miRNA processing gene variants and depression. They genotyped three polymorphisms from three miRNA processing genes (DGCR8, AGO1, and GEMIN4) in a case-control study including 314 patients and 252 matched healthy controls. Frequencies of genotypes and alleles showed significant difference between patients with depression and healthy controls in DGCR8 rs3757 and AGO1 rs636832. An allele frequency was significantly higher in rs3757 and lower in rs636832, respectively. Variant allele of DGCR8 rs3757 was associated with increased risk of suicidal tendency and improvement response to antidepressant treatment, whereas the variant of AGO1 rs636832 showed decreased risk of suicidal tendency, suicidal behavior, and recurrence. Whereas allele frequency showed a significant difference when compared patients with remission to controls, no significant differences were found in GEMIN4 rs7813 between patients and healthy controls. DGCR8 rs3757 and AGO1 rs636832 were found to have significant association with depression, and GEMIN4 rs7813 did not affect susceptibility to depression. These observations suggested that miRNA processing polymorphisms may affect depression risk and treatment.
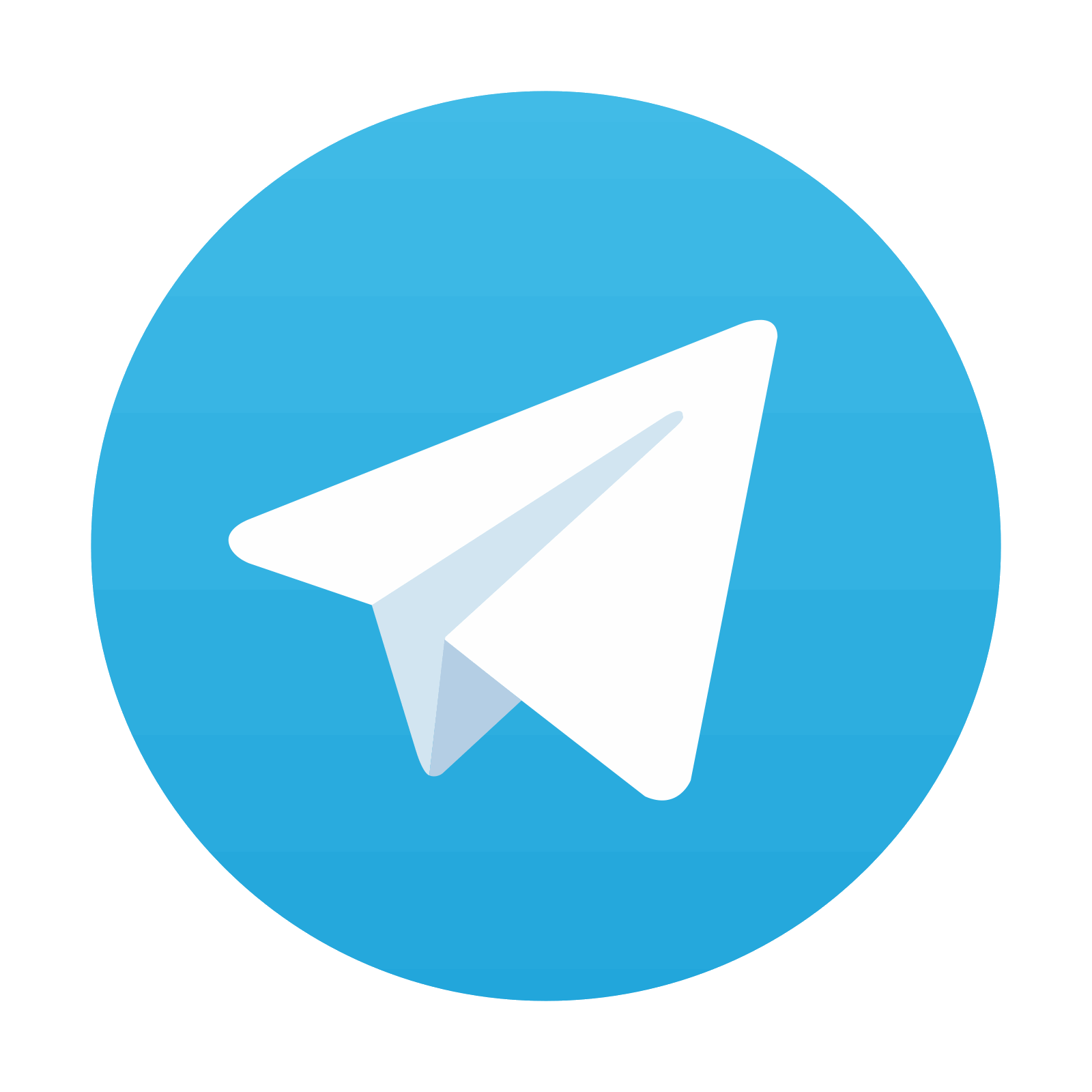
Stay updated, free articles. Join our Telegram channel
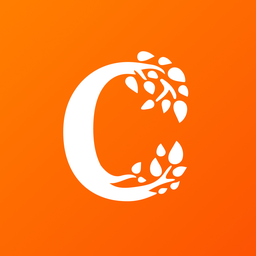
Full access? Get Clinical Tree
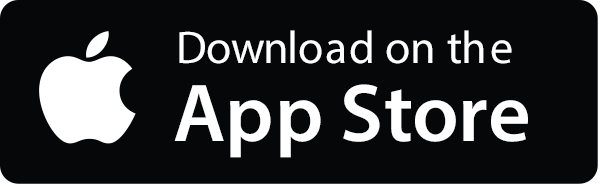
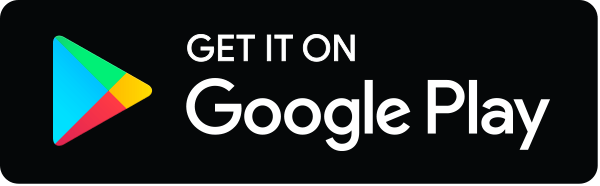