13 Minimizing Ionizing Radiation in Minimally Invasive Spine Surgery
Keywords: ALARA, automatic brightness control, fluoroscopy, inverse square law, radiation exposure
Sit down before facts with an open mind. Be prepared to give up every preconceived notion. Follow humbly wherever and to whatever abyss Nature leads or you learn nothing. Don’t push out figures when facts are going in the opposite direction.
Admiral Hyman G. Rickover
13.1 Introduction
All surgical procedures performed on the spine, minimally invasive or otherwise, require some form of radiographic imaging. Whether that imaging is cross-table radiography, fluoroscopy or intraoperative computed tomography (CT), radiation exposure is the inevitable consequence. Furthermore, in minimally invasive approaches, the absence of widely exposed anatomical landmarks by its very nature mandates another form of visualization. Before computer-assisted navigation, fluoroscopy assumed the role as an alternate form of visualization for spine surgery, but it rapidly took on a central role for minimally invasive spine surgery (MIS). As a result, radiation exposure was greater during minimally invasive procedures than during open procedures. At one point, greater radiation exposure seemed to be an unavoidable aspect of applying minimally invasive techniques. 1
Perhaps the greatest detriment to the increased use of fluoroscopy that accompanied the rise of minimally invasive surgery during the early part of this century was that the increased use did not parallel a proportionate increase in knowledge about the fundamentals of fluoroscopy. There was no concerted effort to increase radiation awareness or training. As mentioned in the previous chapter, I finished a residency without the faintest idea of how a fluoroscope generated radiation or any formal training as to how to adequately protect myself from radiation. I was never issued a dosimeter. I have no idea as to the amount of radiation I was exposed to throughout my residency. Wasn’t a lead gown all I needed?
Fluoroscopes are now ubiquitous both in residency training and in practice. Dosimeters are not. Although surgeons have acknowledged the greater radiation exposure with minimally invasive procedures, only recently has there been an appreciation for radiation exposure during residency training. 2 I noted my concern about the greater amount of radiation exposure associated with minimally invasive techniques at the beginning of Chapter 12. The current body of medical literature would certainly support that concern. The question now becomes what can we do about it.
My focus for this chapter is twofold. First, I want to discuss a framework in which you can decrease your exposure to radiation when you perform surgical procedures requiring a fluoroscope. Second, I would like to present alternate techniques for the fluoroscopic unit that will decrease the amount of radiation exposure. Chapter 12 provided the fundamentals of fluoroscopy that will make understanding alterations in voltage and current settings within the X-ray tube understandable. My overarching goal is for the pendulum to begin to swing in the other direction. I want surgeons to relinquish the idea that a minimally invasive technique necessitates more radiation exposure. I want the spine surgeons of tomorrow to replace that notion with the concept that they can perform minimally invasive surgical procedures with less radiation exposure than ever before.
This final chapter began with a quote from Admiral Hyman G. Rickover, the father of the nuclear navy. When the topic is radiation exposure and awareness, there may not be a better authority than Rickover. Again, it was my all-to-brief service in the nuclear navy as a diving medical officer and radiation health officer that led directly to my interest in the radiation exposure that can occur in minimally invasive approaches. Admiral Rickover transformed the diesel-powered U.S. Navy into a fleet of nuclear-propelled submarines and aircraft carriers. Perhaps his greatest legacy is the number of nuclear reactor accidents that have occurred among the 200 nuclear submarines and 23 nuclear-propelled aircraft carriers and cruisers commissioned since the birth of the nuclear navy with the launch of the U.S.S. Nautilus in 1954. That number is zero. Admiral Rickover created a culture of caution, awareness and precision when dealing with radiation and nuclear power. Perhaps grafting elements of Rickover’s culture into MIS would be transformative. In previous chapters throughout this Primer, I examined the experience of master surgeons from decades ago to establish a basis for the various minimally invasive procedures we perform today. In a similar manner, I would like to reference Admiral Rickover’s management objectives as a framework for a minimally invasive spine surgeon’s work with radiation.
Rickover’s management objectives:
Require rising standards of adequacy.
Be technically self-sufficient.
Face facts.
Respect even small amounts of radiation.
Require adherence to the concept of total responsibility.
Develop the capacity to learn from experience.
Over the years, I have often reflected on the admiral’s quote that began this chapter as I listened to some of my colleagues disparage the importance of radiation awareness and summarily dismiss my efforts to minimize exposure. All the while, we are all becoming increasingly able to name spine surgeons who died too soon of cancer, including, most recently, one of my mentors from medical school who initially interested me in the study of the spine. I will readily concede that correlation does not imply causation, but it has become more and more difficult for me to dismiss the mounting evidence that there may be more to this story. The last sentence of Rickover’s quotation has always lingered in my mind, “Don’t push out figures when facts are going in the opposite direction.” I saw my dosimeter readings from my time in a nuclear submarine juxtaposed with my dosimeter readings from when I performed minimally invasive spine procedures next to a fluoroscope. As much as I initially wanted to dismiss this inconvenient and pestering element of MIS, I found that I could not. I believe that when it comes to MIS and radiation exposure, we must heed the admiral’s advice and follow the facts in the direction they are heading. We should respect even small amounts of radiation. We should push nothing aside, whether convenient or inconvenient to do so. We should strive to make ourselves technically self-sufficient with our understanding of fluoroscopy. Collectively, these efforts will raise the standards of adequacy regarding radiation exposure in MIS. The contents of this final chapter represent my lifelong efforts to adhere to the admiral’s sage words.
13.2 As Low as Reasonably Achievable: The ALARA Principle
There is no safe dose of radiation. For this reason, the International Commission on Radiological Protection (ICRP) introduced a systematic approach to limit the radiation exposure to persons working with radiation. Our profession in MIS places us firmly in the category of radiation workers. Instead of focusing on a number or a dose, the central principle recommended by the ICRP is for each encounter with radiation to be as low as reasonably achievable (ALARA). The ALARA principle comprises three core elements: dose limitation, dose justification and dose optimization. Applying the ALARA principle in every surgery involving fluoroscopy is a central tenet for minimizing your exposure to ionizing radiation in spine surgery throughout your career.
13.3 Dose Limitation
13.3.1 The Inverse Square Law
When you strike the middle C key on a piano in an empty room, the sound waves travel outward from the source in all directions. The closer to the piano that you are standing, the louder the sound will be; the farther you are from the piano, the softer the sound. As those sound waves travel through space away from the piano, a geometric dilution occurs that results in the softer sound. If you double your distance from the piano, the intensity of the middle C note will decrease to one-fourth. If you halve the distance you are standing from the piano, the sound of that key stroke will have four times the intensity. The law that governs this phenomenon is known as the inverse square law:
where r is the radius of a sphere and equal to the distance from the source.
This law of physics governs not only sound but also light and radiation. Therefore, the intensity of radiation is inversely proportional to the square of the distance from the source of the radiation. When the X-ray tube of a fluoroscope emits radiation for an image in surgery, the radiation travels through space from that point source just like the sound of the middle C key from the piano in the empty room. Geometric dilution occurs in an isotropic fashion as the radiation travels through space ( ▶ Fig. 13.1). The intensity of that radiation plummets as the distance from the source increases. The inverse square law is perhaps the most important principle you need to embrace when considering dose limitation. Whenever possible, always distance yourself as much as possible from the radiation source.
Fig. 13.1 Illustration showing the geometric dilution that occurs with radiation as the distance from the X-ray source increases. At three times the distance from the source, the dose of radiation is one-ninth of the exposure at the source. Standing on the side of the table next to the X-ray tube exposes the surgeon to a higher dose of radiation than standing on the side of the table next to the image intensifier. The inverse square law is one of the central tenets to follow to minimize your exposure to ionizing radiation throughout your career.
Radiation-Protective Apparel
At this point in the chapter, you might find it surprising to learn that the most important aspect of dose limitation is not wearing your apron or thyroid shield but wandering as far away as possible from the X-ray tube as it acquires an image. Still, the inverse square law does not supplant the importance of radiation protective apparel. Wearing radiation-protective gowns and thyroid shields is always inherent to dose limitation. Note that I have intentionally refrained from using the term lead, which has become the operating room vernacular for radiation-protective gowns and shields. In the interest of preserving the spines of persons who need to wear such apparel for hours at a time, manufacturers have developed lighter alternatives to leaded gowns, most of which contain no lead at all. Whether or not your radiation-protective gown contains lead, there are few better investments to make than to purchase your own radiation-protective gear, including a pair of radiation-protective glasses.
13.4 Dosimetry
I completed my residency without the faintest idea about the extent of my radiation exposure throughout my years of training. At that time, residents in my training program were not issued dosimeters. However, had it not been for the dosimeter the Navy issued me upon my arrival at the Naval Hospital in San Diego, I would never have been able to juxtapose those dosimetry readings with my dosimetry readings aboard the U.S.S. Buffalo, a fast-attack nuclear submarine. Without that glaring difference in those data points, my concern about radiation exposure would never have arisen. In the absence of those data, I would never have conceived of a need to compose the final two chapters in this Primer. A dosimeter provides valuable data regarding radiation exposure. Knowledge of the data increases awareness and potentially changes behavior. Every surgeon exposed to radiation, whether in training or in practice, should have access to a dosimeter. The decision to wear and monitor that dosimeter is ultimately left to the surgeon, but the capacity to monitor exposures must be there.
13.5 Collimation
In Chapter 12, I explained how an X-ray tube generates X-rays that then make their way through the patient to the image intensifier to generate an image. What I did not explain is that those X-rays that leave the X-ray tube do not all make it across the patient. In fact, they have one of three fates: they will pass directly through the patient, they will become completely absorbed by the patient or they will interact with tissue and bone to reflect and scatter. Those X-rays that pass directly through the patient with no interaction are directly responsible for contributing to the image of the spine that appears on the screen. The appropriate title assigned to these X-rays is image-forming X-rays. Obviously, the X-rays that are completely absorbed by the patient contribute nothing to the image. The X-rays that reflect and scatter, a phenomenon known as Compton interaction, take away from the image. Scattered X-rays decrease the contrast and result in a grainy appearance to the image. Even more important is that the reflected and scattered X-rays contribute to the radiation exposure to the surgeon and operating room staff. That radiation exposure is obviously greatest for persons standing on the side of the X-ray tube where the beams reflect from the patient immediately onto the surgeon and other operating room staff; it is significantly less for persons standing on the side of the image intensifier ( ▶ Fig. 13.2).
Fig. 13.2 The Compton effect. As X-rays interact with matter, they have one of three fates: (1) they pass through the patient and contribute to an image of the spine, (2) they become absorbed by the patient or (3) they strike the patient and scatter. The illustration demonstrates the Compton scatter of X-rays that can contribute to a surgeon’s radiation exposure. Scattered X-rays provide no useful information on the radiograph but instead reduce the image contrast. Compton scatter is yet another reason the radiation exposure is highest on the side of the X-ray tube.
In a perfect world, only the X-rays that pass through the patient with no interaction would be the ones that reach the image intensifier. But complete absorption and Compton scatter are an inevitable consequence of X-rays interacting with matter. Thus, we are faced with creating an environment wherein we optimize image-forming X-rays that contribute to the image while minimizing scattered X-rays that take away from the image. One way to optimize the image-forming X-rays and to minimize the scattered X-rays is to narrow the field of radiation. The term used to describe this is collimation.
Collimation is the process by which shutters within the fluoroscope narrow the field of view, analogous to an aperture on a camera limiting the light that enters the lens ( ▶ Fig. 13.3). The result of collimating an X-ray beam is twofold. First, a collimated X-ray beam decreases the surface area of the patient exposed to radiation, sparing unnecessary exposure to adjacent tissue. Second, the less of the patient’s volume that is exposed to the X-ray beam, the less scatter there will be of X-ray incident to the detector. Reducing Compton scatter improves image contrast. The net result is less radiation exposure to the patient, less X-ray scatter to the surgeon and operating room personnel and a high proportion of image-forming X-rays. Collimating the X-ray beam will result in less radiation and a higher-quality image. As a rule, I always collimate to the smallest field of view for the segment. The capacity to collimate an image is available in almost all commercially available fluoroscopes.
Fig. 13.3 Collimation of the useful X-ray beam. Shutters within the X-ray tube of the fluoroscope are used to decrease the exposure to adjacent tissues. (a) The illustration shows the decrease in Compton scatter. The reduced Compton scatter decreases radiation exposure on the side of the X-ray tube. The result is a higher-quality image at a lower radiation dose. (b) A lateral fluoroscopic image of a minimally invasive lumbar fusion without collimation. (c) The same lateral fluoroscopic image using a collimated beam. The reduction in Compton scatter sharpens and brightens the image while decreasing radiation exposure to the surgeon from the scatter off the patient. Both images were acquired at the same kVp and mA.
13.6 Justification
Confirmation of the operative segment is one instance in which the fluoroscopic image is the only modality that can provide the information needed to move the operation forward. However, subsequent images are of limited value. I have watched residents and fellows acquire image after image when doing nothing more than passing a dilator onto the spine at the same location for a microdiscectomy, a laminectomy or an instrumented fusion, whereas one image of the initial dilator was all that was needed until the insertion of the minimal access port. Those additional images were unnecessary because they provided no actionable information to justify taking them. I have also watched those same residents and fellows request an image with every quarter-turn of the tap. When I ask them what it is they are looking for, I have yet to hear a satisfactory answer. Once you have probed a pedicle and established a trajectory into that pedicle with a fluoroscopic image, no further imaging is necessary until the threads of the tap are buried and you would like to determine the optimal length for the pedicle screw. As noted in Chapter 4 on transforaminal lumbar interbody fusion (TLIF), after capturing an image with the instrument in the ideal position, I maintain that position relative to the blades of the minimal access port as the instrument advances. If that position remains unaltered, subsequent images do not provide actionable information. However, an image with the threads buried will provide valuable information to help you determine the length of the pedicle screw that is needed. Images of the tap advancing along the same trajectory through the pedicle are of limited value and are not in keeping with the justification component of the ALARA principle.
13.7 Percutaneous Pedicle Screws
The inability to directly visualize the anatomy while placing pedicle screws percutaneously mandates imaging at every step of the placement. When computer-assisted navigation guides the percutaneous placement of a pedicle screw, radiation exposure to the surgeon and the operating room staff becomes a moot point. However, in the absence of image guidance, radiation exposure begins to rise. The published literature documents a range of 22 to 29 seconds of fluoroscopy with a dose of up to 10.3 mREM per percutaneous screw in experienced hands.3,4 Furthermore, the very nature of the procedure mandates the surgeon’s proximity to the X-ray source, holding instruments, thereby limiting the capacity to harness the inverse square law to the surgeon’s advantage. The inevitable consequence is exposure to greater amounts of radiation exposure for fluoroscopic placement of percutaneous pedicle screws compared to that for pedicle screw placement using direct visualization of anatomical landmarks with intermittent fluoroscopic guidance. Coupled with the inability to directly visualize the facets for a Smith–Petersen osteotomy or the transverse processes for a posterolateral fusion, this greater radiation exposure has made it increasingly difficult for me to use percutaneous screws for single-level and two-level fusions.
13.8 Optimization
In 1984, Wesenberg and Amundson 5 published one of the first articles detailing a low-dose protocol for the use of fluoroscopy. Their focus was the radiation exposure in children undergoing diagnostic imaging. At the root of their concern were the radiation doses and fluoroscopy times required for specific procedures (e.g., cardiac catheterization, cystourethrograms and upper gastrointestinal evaluations). Wesenberg and Amundson wanted to reduce these radiation exposures because of the inherent risk of radiation causing subsequent malignancies in growing children. Their 1984 manuscript begins with a thoughtful sentence that is still as relevant today for MIS as it was then for pediatric imaging: “the goal of diagnostic radiology is to make the most accurate diagnosis with the least amount of radiation and risk to the patient … this is particularly applicable to pediatric radiology.”5
Over the years, subsequent authors continued to develop techniques to minimize radiation exposure while still providing adequate diagnostic imaging for children. The pediatric cardiology literature was especially focused on this topic.6,7 What is striking as one juxtaposes the pediatric radiology literature and the MIS literature during the same period is how diametrically opposed were the trajectories on the same topic. Pediatric radiologists kept documenting remarkably reduced exposures, whereas minimally invasive spine surgeons kept publishing reports of the exact opposite.3,6,7,8,9 As the concern about radiation exposure in minimally invasive spinal procedures became a barrier to the application of the techniques, taking a page out of the pediatric radiology playbook was the logical step forward. In fact, when spine surgeons finally applied the well-honed fluoroscopy protocols from the pediatric radiology literature, radiation exposure and fluoroscopy times both decreased significantly without compromising the safety of the procedure.10,11
The remainder of this chapter focuses on exploring these low-dose protocols in the context of the final element of the ALARA principle: optimization. In the upcoming pages, I will discuss the techniques that pediatric radiology has used for decades to decrease radiation exposure with fluoroscopy. Applying these protocols will require an understanding of the types of fluoroscopy and especially the importance of acquisition time. Incorporating your understanding of peak kilovoltage (kVp) and milliampere (mA) into these protocols will serve as a background to help you harness these parameters to your advantage. You will discover that doing nothing more than altering the fluoroscopic technique or manually adjusting the kVp and mA will have a direct impact on both the radiation exposure required for each image and the quality of that image. In imaging the spine for minimally invasive spine procedures, we would do well to adopt the same attitude that Amundson and Wesenberg had more than three decades ago toward pediatric patients. Striking a balance between radiation exposure and image quality is the essence of optimization.
13.9 Types of Fluoroscopy
Let us return to the operating room, where you are waiting for the fluoroscopy technologist to turn on the fluoroscope so that you can begin a procedure. When the technologist finally plugs in the fluoroscope and turns it on, software begins to load in the central processing unit (CPU) and the thoriated tungsten filament begins to warm up, preparing for the surge of voltage coming its way. As the progress arrows populate the screen, the fluoroscopy algorithms are loaded. These are written in a manner so that when it is finally time to hit the button and obtain an image, the kVp and mA will automatically be determined such that the result will be an optimal image with ideal brightness and contrast. The type of fluoroscopy that accomplishes this ideal image is known as continuous interlaced fluoroscopy, or just continuous fluoroscopy. It is the default setting that happens simply by turning on the fluoroscope. In all likelihood, this setting is the one you have been using all along for your operations.
Continuous fluoroscopy offers a reliable image without having to manipulate any of the parameters. As the name implies, the X-ray beam is continuous. When the technologist pushes the button, that continuous X-ray beam generates 30 images per second that become interlaced to create the composite image. The kVp and the mA are initially determined by an algorithm within the CPU. The automatic brightness control (ABC) further adjusts these parameters using the patient’s size through a feedback mechanism. A sensor inside the image intensifier monitors the brightness. When the brightness of the image is inadequate because of the size of the patient, the ABC algorithms increase the kVp first. The result is an increase in the number of projectile electrons striking the tungsten target, which, in turn, increases the number of diagnostic X-rays. The net increase in the number of higher-energy X-rays results in a greater proportion of X-rays that can better penetrate the body and serve as image-forming X-rays. The cervical spine has narrower dimensions than the lumbar spine, so the need for penetration is less. As a result, imaging in the cervical spine requires a lower kVp.
When you consider an acquisition time that ranges from 1 to 3 seconds per image, the total fluoroscopy time and exposure have the potential to quickly add up. For example, if you were to obtain 40 images throughout an instrumented lumbar fusion case using this technique, fluoroscopy times would range from 40 to 120 seconds, which is well within the reported range of fluoroscopy times for minimally invasive fusions.4,8,12Many authors have understandably considered that amount of radiation exposure to be a liability and, for obvious reasons, it has become one of the main criticisms of minimally invasive techniques. One approach to dealing with radiation is to use computer-assisted navigation, which can decrease radiation exposure.3 Another approach is to use the alternative fluoroscopy technique introduced by pediatric radiologists. Fully embracing that decades-old technique alone can quickly dispel those criticisms.
13.10 Pulsed Fluoroscopy
The less time the X-ray beam is on, the less radiation exposure will occur to the patient, the surgeon and the operating room personnel. Two simple modifications to the settings on the fluoroscope will accomplish this task. The first step is to turn off the ABC. The absence of this control eliminates the feedback loop and the additional seconds of radiation exposure that are required to complete the loop. However, it also excludes the algorithm that automatically determines the ideal kVp and mA to optimize the image. Disabling the ABC is equal parts asset and liability. Every image will be acquired at the default kVp and mA settings. The drawback may be the need to manually increase or decrease these values to view an adequate image. The advantage is a substantial decrease in radiation exposure. Working closely with your radiology technologist to determine these settings will allow you to acquire an adequate image at the lowest amount of radiation exposure.
The second modification is to intermittently turn off the X-ray beam during image capture. Instead of having a continuous beam for image capture, turning the X-ray beam on and off will decrease the amount of radiation exposure. Such a setting is known as pulsed fluoroscopy ( ▶ Fig. 13.4). X-ray beam pulsation obviously decreases radiation exposure, but it also decreases image quality. Two key parameters guide X-ray beam pulsation: pulse width and pulse rate. The pulse width is the duration of the pulse itself, specifically how long the X-ray beam is on for image capture before turning it off. A shorter pulse width has the obvious advantage of less radiation, but it also limits the number of X-rays that contribute to image formation. The fewer the number of image-forming X-rays penetrating a patient, the lower the quality of the image and the lower the radiation exposure.
Fig. 13.4 Conventional fluoroscopy compared to pulsed fluoroscopy. (a) Lateral fluoroscopic image at the completion of a lumbar fusion captured at 118 kVp and 5.48 mA using conventional fluoroscopy. Conventional fluoroscopy acquires 30 frames per second to create the composite image. Acquisition time for this image was 1.2 seconds, indicating that more than 30 frames contributed to the image. (b) Graphical representation of radiation exposure when using conventional fluoroscopy. (c) A pulsed fluoroscopic image of the same region of the spine obtained at 114 kVp and 2.44 mA with a pulsed X-ray beam. Although the quality of the image is lower, the resolution of the anatomy is more than adequate for instrumentation and interbody placement. The acquisition time was 0.15 seconds. Pulsed fluoroscopy produced adequate images with less radiation in one-tenth of the time required for conventional fluoroscopy. (d) Graphical representation of radiation exposure when using pulsed low-dose fluoroscopy.
The pulse rate is the number of fluoroscopic image frames that are generated per second. For imaging a static subject, such as the spine, the pulse rate is less important. High pulse rates are necessary to study anatomy in motion, such as the assessment of diaphragmatic motion, a cardiac valve, or esophageal motility. The phenomenon known as temporal resolution is crucial for studying anatomy in motion. Since our subject matter is the motionless spine, there is little need for temporal resolution. Therefore, lowering the pulse rate will not have a significant effect on the image of a static subject. Applying the pulsed fluoroscopy setting will dramatically reduce radiation exposure for surgical procedures on the spine.10 The combination of turning off ABC with pulsed fluoroscopy will further reduce radiation exposure by decreasing acquisition time in addition to the dose per frame. The trade-offs for these low doses and short acquisition times are the quality and the sharpness of the image. The concepts of pulse width and pulse rate are demonstrated in the accompanying video (Video 13.1).
13.11 Acquisition Time and the Digital Spot Technique
An analogous scenario to continuous fluoroscopy and even to pulsed fluoroscopy would be taking a photograph using your smartphone. Instead of the photo option, you select the video option. With activation of the video capture, the camera begins by focusing on the subject. The sensors provide feedback mechanisms within the smartphone camera to adjust the brightness and the contrast of the image before finally rendering the perfect image. Over the span of 3 seconds of video, the very last few milliseconds have perfect contrast, brightness and focus. Continuous interlaced fluoroscopy renders an image in a similar manner. The reality is that we do not use most of the footage. The final frame is all that we actually view, but the first few seconds are necessary because of the process of acquiring the image. The adjustments that occur in brightness, contrast and focus occur in the first few moments of image acquisition. For that single image, the acquisition time was quite long, and the number of frames captured for that final few milliseconds of footage was quite high. The logical question is why we would want to acquire a static image in this manner. Why would we not use the photo option with the instantaneous acquisition of a single frame?
Remarkably, the process described earlier is exactly how most of us use conventional fluoroscopy as we operate on the spine. Images created with continuous fluoroscopy default to the “last image hold,” which is the image that remains on the screen when the ray beam turns off. That image is the integration of multiple frames. The radiation exposure for that last image hold is the number of frames times the radiation exposure for the acquisition of those frames.13 In reality, we are capturing hundreds of unnecessary frames in a video format to obtain one static composite image at the end of a feedback mechanism. Along the way, we are increasing our exposure to radiation. Instead, our goal should be to capture an image of the spine with the fewest frames and with the shortest possible acquisition time. Thus, our real goal should be to take an image of the spine with a photo setting instead of a video setting.
However, there is a reason why fluoroscopy obtains 30 frames per second to generate an image. The amount of noise in an image is inversely proportional to the square root of the number of photons used to acquire that image. As noted in Chapter 12, the number of photons that create an image is proportional to the number of diagnostic X-rays striking the image intensifier. Because of the internal lag characteristics of the human eye–brain response, a real-time sequence of an image generated at 30 frames per second is perceived with much less noise than a single static fluoroscopic image. In other words, the composite image of three to five frames of fluoroscopy will appear sharper and brighter than a single frame of fluoroscopy.
Digital spot imaging solves the problem of acquisition time and the need to create a composite image ( ▶ Fig. 13.5). It is the equivalent of taking a photograph with the photo setting instead of the video setting. Digital spot images are immediate exposures, but the consequence of taking a single exposure is that the process requires a much higher dose of radiation than that required for a single frame of fluoroscopy. However, it is a single frame captured at a low acquisition time (typically, 0.15 seconds) with much better image quality and resolution than a pulsed fluoroscopy image. Although there is a considerable amount of radiation in a single digital spot, it must be balanced with the number of frames acquired to have an equivalent view of the anatomy with fluoroscopy. When viewed in that context, digital spot images can compare quite favorably, especially when a sharper image is needed for instrumentation.
Fig. 13.5 Conventional fluoroscopy compared to digital spot fluoroscopy. (a) The same lateral conventional fluoroscopy image from▶ Fig. 13.2 at the completion of a lumbar fusion captured at 118 kVp and 5.48 mA. Conventional fluoroscopy acquires 30 frames per second to create the composite image. Acquisition time for this image was 1.2 seconds, which indicates that more than 30 frames contributed to the image. (b) The same lateral fluoroscopic image obtained with a digital spot technique. Acquisition time was 0.15 seconds, with 120 kVp and 21.60 mA. Although the dose is significantly higher, the image is captured as a single frame. These two images are almost indistinguishable in brightness, contrast and sharpness. However, the acquisition time is significantly shorter for the digital spot technique.
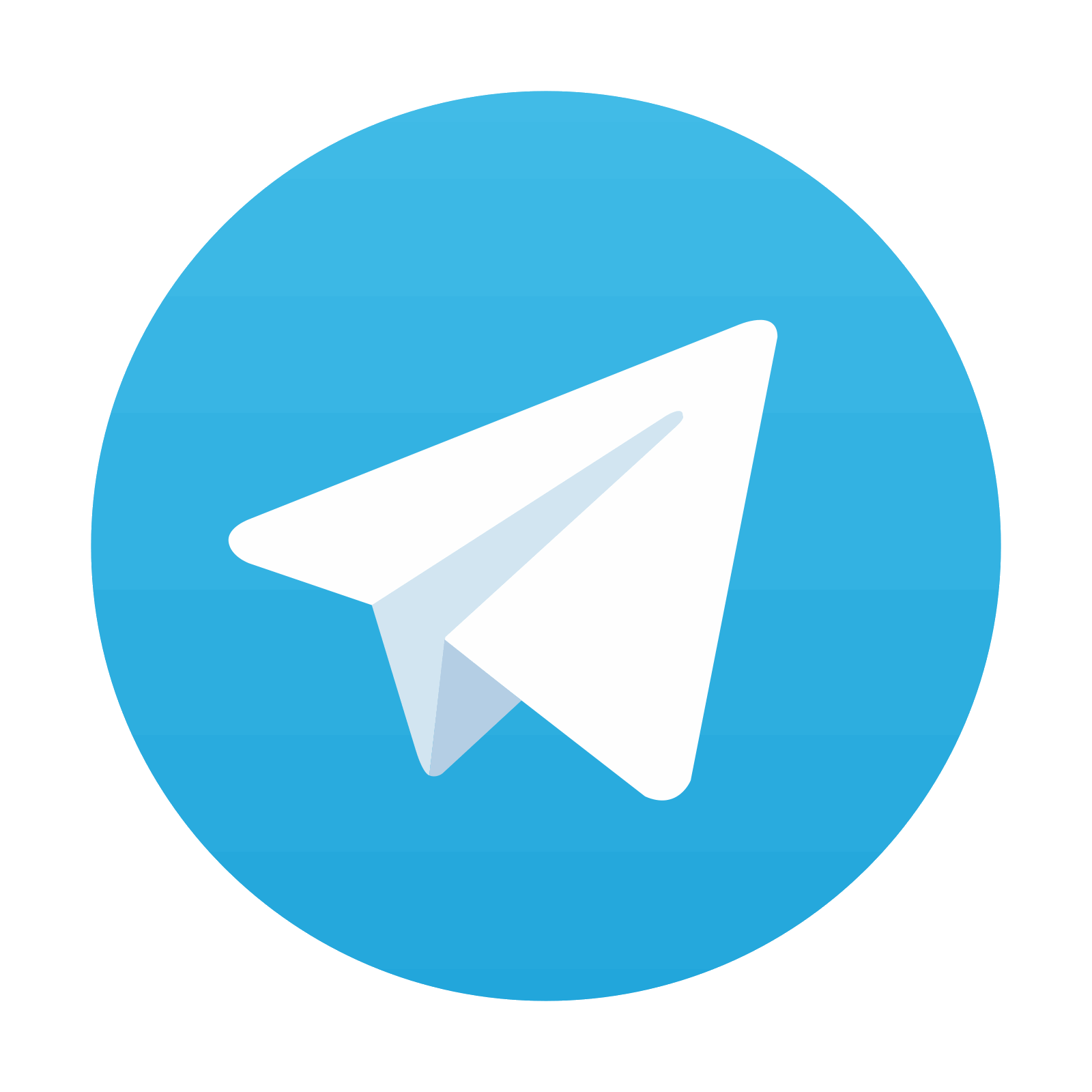
Stay updated, free articles. Join our Telegram channel
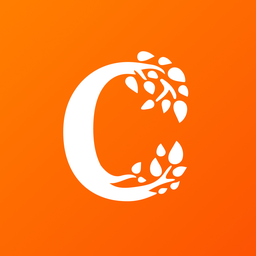
Full access? Get Clinical Tree
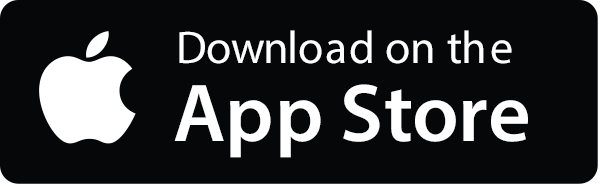
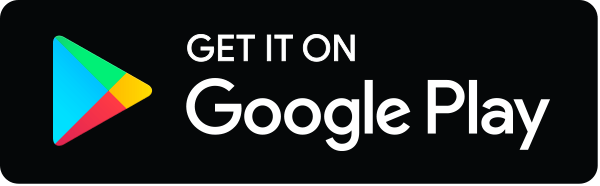