Mitochondrial myopathies and neuropathies or neuromyopathies refer to a heterogeneous group of disorders caused by dysfunction of mitochondria.1–10 Mitochondrial disorders can be classified according to the associated biochemical, genetic defects, or clinical phenotype (Tables 30-1,30-2,30-3). One difficulty in classifying patients by any particular scheme is the clinical-phenotypic heterogeneity associated with specific mitochondrial mutations and the genetic heterogeneity in well-defined clinical phenotypes that are seen with mitochondrial disorders.
Metabolic Function | Defects |
---|---|
Substrate transport | Carnitine palmitoyltransferase (CPT) |
Primary systemic/muscle carnitine deficiency | |
Secondary carnitine deficiency | |
Combined carnitine and CPT deficiency | |
Substrate utilization | Pyruvate decarboxylase deficiency |
Pyruvate dehydrogenase deficiency | |
Pyruvate carboxylase deficiency | |
Fatty acid β-oxidation defects | |
Kreb cycle | Fumarase |
α-Ketoglutarate dehydrogenase deficiency | |
Dihydrolipoyl dehydrogenase | |
Oxidation/phosphorylation coupling | Luft’s syndrome: Loose coupling with hypermetabolism |
Respiratory chain | Complex I |
Complex II | |
Complex III | |
Complex IV | |
Complex V | |
Combinations of I–V |
|
Disease | Mode of Inheritance | Mitochondrial DNA Mutation | Gene Location |
---|---|---|---|
Kearns–Sayre syndrome | Sporadic | Single large mtDNA mutation | Large area of mt genome |
PEO | Sporadic | Single large mtDNA mutation | Large area of mt genome |
PEO | Maternal | Point mutations of mtDNA | tRNALeu, tRNAIle, tRNAAsn |
PEOA | Autosomal dominant | Multiple mtDNA deletions | POLG1, C10orf2 (twinkle); less common: ANT1, POLG2, TK2, OPA1, DGOUK, RRM2B |
PEOB | Autosomal recessive | Multiple mtDNA deletions | POLG1; less common: TK2, DGOUK, RRM2B, MPV17, DNA2, SGP7 |
ARCO | Autosomal recessive | Multiple mtDNA deletions | Unknown nuclear gene |
MERRF | Maternal | Point mutations of mtDNA | tRNALys, tRNALeu, tRNAHis, tRNAPhe, tRNASer, MTND5 |
MERRF | Autosomal recessive | Multiple mtDNA deletions | POLG1 |
MELAS | Maternal | Point mutations of mtDNA | tRNALeu, tRNAVal, tRNALys, tRNAPhe, tRNASer, ND5, ND4, ND1, MTCYB |
MNGIE | Autosomal recessive | Multiple mtDNA deletions | TYPM, POLG1, RRM2B |
MNGIE | Maternal | Point mutations of mtDNA | tRNALys |
Leigh syndrome | Maternal | Point mutations of mtDNA | MTND3, MTND5, MTND6, MTCO3, MTATP6, tRNAVal, tRNALys, tRNATrp, tRNALeu |
Leigh syndrome | Autosomal recessive | None | NDUFV1, NDUFS1, NDUFS3, NDUFS4, NDUFS7, NDUFS8, SDHA BCS1L, COX10, COX15, SCO2, SURF1, LRPPRC |
Leigh syndrome | X-linked | None | PDHA1 |
Leigh syndrome | Sporadic | Single large mtDNA mutation | Large area of mt genome |
Recurrent myoglobinuria | Sporadic or autosomal recessive | Mutations and microdeletions of mtDNA | MTCO1, 2, and 3, MTCYB, ND4 |
Recurrent myoglobinuria | Maternal | Point mutations of mtDNA | tRNAPhe |
MLASA | Autosomal recessive | None | PUS1 |
SANDO | Autosomal recessive | Multiple mtDNA deletions | POLG1, C10orf2 |
SANDO | Autosomal dominant | Multiple mtDNA deletions | C10orf2 |
Navajo neurohepatopathy | Autosomal recessive | None | MPV17 |
Optic atrophy 1 | Autosomal recessive | None | OPA1 |
The mitochondria are responsible for converting fuels (carbohydrates, lipids, and proteins) into energy for the cells. Fatty acids are metabolized into molecules of acetyl-CoA within the mitochondria. Amino acids are converted to pyruvate in the mitochondria. Carbohydrates are metabolized to pyruvate in the cytoplasm and then transported into the mitochondria. Pyruvate from either source is converted into acetyl-CoA. Acetyl-CoA, then enters into the Krebs cycle from which electrons are generated. Electrons derived from the Krebs cycle are shuttled to the respiratory chain and processed through complexes I–V to generate ATP molecules. Thus, mitochondrial disorders can be classified according to the metabolic defect present (1) transport, (2) substrate utilization, (3) Krebs cycle, (4) oxidation/phosphorylation coupling, and (5) respiratory chain (Table 30-1).
Some of the biochemical abnormalities seen in various mitochondrial disorders are nonspecific and the result of primary “upstream” defects in metabolic pathways. For example, cytochrome oxidase (COX) deficiency is seen in many types of mitochondrial myopathy and does not imply that the primary mutation lies in one of the genes encoding for subunits of COX. The rapid advances of molecular genetics may provide a better classification scheme. The mitochondrial disorders may be classified by their genetic defect (1) mitochondrial DNA (mtDNA), (2) nuclear DNA (nDNA) mutations directly or indirectly affecting the mitochondrial respiratory chain complex, or (3) nDNA mutations that are involved in mtDNA maintenance or mitochondrial dynamics (Table 30-2; Fig. 30-1).1–6 Notably though, there is significant phenotypic variability even in patients with the same genetic mutation. Therefore, a combined classification scheme is currently favored because of phenotypical variability and problems inherent in current genotyping capabilities (Table 30-3). Prior to discussing specific disorders, we will review a few basic principles regarding the mitochondrial genome and different inheritance patterns of mitochondrial disorders.
Figure 30-1.
Genes associated with mitochondrial disease. Mutations have been identified in genes encoding CI, CII, CIII, CIV, and CV subunits and assembly factors; genes involved in mitochondrial DNA (mtDNA) maintenance (via nucleotide metabolism), mtDNA replication, and mtDNA expression; genes affecting the electron carriers coenzyme Q (CoQ) and cytochrome c (CytC); genes affecting FeS assembly; and genes involved in protein import, toxicity/apoptosis, and membrane function. Recently identified genes described in this review are highlighted in red. Genes affecting oxidative phosphorylation for which mutations are not reported to cause neuropathology are underlined. rRNA, ribosomal RNA; tRNA, transfer RNA. Currently, most cases of mitochondrial encephalopathy are untreatable, other than by relieving certain symptoms. Therefore, there is a great need to better understand the genetics of mitochondrial disease, which will enable prenatal diagnoses and will deliver the deeper understanding of mitochondrial function needed for the development of effective therapies. Recent advances in sequencing technology indicate that we may be on the cusp of a revolution in the way genetic diseases, such as mitochondrial encephalopathy, are diagnosed. (Reproduced with permission from Tucker EJ, Compton AG, Thorburn DR: recent advances in the genetics of mitochondrial encephalopathies. Curr Neurol Neurosci Rep. 2010;10(4):277–285.)

The mitochondrial genome comprises 16.5-kB circular double-stranded DNA that contains no noncoding regions (i.e., introns). In fact, contiguous mitochondrial genes overlap in some areas. There is a single promoter site, and transcription is polycistronic such that mitochondrial genes are transcribed as two large RNAs. These are subsequently cleaved into 13 respective messenger RNAs (mRNA), 2 ribosomal RNAs (rRNA), and 22 transfer RNAs (tRNA). Interestingly, the genetic code for translation of human mitochondrial genes differs from the standard code which governs the translation of human nuclear genes.
The 13 mRNAs are translated into 13 polypeptides that are subunits of the respiratory chain complexes. Also note that any mutation in a mitochondrial tRNA gene can impair the proper translation of the 13 mitochondrial mRNAs. Importantly, the 13 proteins encoded by the mitochondrial genome account for less than 5% of all mitochondrial proteins. Thus, the majority of mitochondrial proteins are encoded by the nuclear genome that are translated in the cytoplasm and subsequently are transported into the mitochondria. Furthermore, the nucleus appears to regulate replication of the mitochondrial genome.
The respiratory chain comprises five multienzyme complexes (complexes I–V) (Fig. 30-2). Complex I (NADH-CoQ reductase) contains 45 subunits, seven encoded by mtDNA; complex II [succinate dehydrogenase (SDH) CoQ reductase] comprises four subunits, each encoded by nuclear genes; complex III (CoQH2-cytochrome c reductase) comprises 11 polypeptide units, one of which is encoded by mtDNA; complex IV (cytochrome c oxidase) has 13 subunits, three encoded by mtDNA; and complex V (ATPase synthetase) comprises 19 subunits, two encoded by mtDNA (Table 30-4).
Figure 30-2.
Schematic view of the respiratory chain. This diagram shows the number of subunits encoded by mitochondrial DNA (mtDNA) and nuclear DNA (nDNA) for each complex. All of the subunits for complex II are encoded by nDNA. Electrons (e−) flow down the respiratory chain and protons (H+) are pumped from the matrix to the intermembranous space through complexes I, III, and IV, then back into the matrix through complex V (ATPase synthase). Cytochrome c (Cyt c) and coenzyme Q (CoQ) are electron carriers. This process generates adenosine triphosphate (ATP). (Reproduced with permission from Walsh RJ. Metabolic Myopathies. Continuum. 2006;12(3):76–120.)

A population-based study in Northern England found that 6.57 per 100,000 adults have a mitochondrial disease and 12.48 per 100,000 of children and adults are at risk for developing a mitochondrial disorder on the basis of identifiable mtDNA mutations.11 Because this study included only disorders caused by mtDNA mutations and not nDNA mutations affecting mitochondria, the prevalence of mitochondrial disorders is certainly higher. Remember that during fertilization, all the mitochondria are contributed by the mother. Hundreds of mitochondria are present in most cells in the body and every mitochondrion has several copies of mtDNA. Mutations involving mtDNA are more common and more likely to manifest clinically than mutations in nuclear genes because of the lack of introns and decreased DNA repair mechanisms in the mitochondrial genome. mtDNA mutations are randomly distributed in subsequent generations of somatic cells during mitosis and germ cells during meiosis. Therefore, some cells will have few or no mutant genomes (normal homoplasty), some will have a mixture of mutant and normal or wild-type mtDNA (heteroplasty), and some will have predominantly mutant genomes (mutant homoplasty). Phenotypic expression depends on the relative proportion of mutant and wild-type mitochondria within each cell within a given organ system. When the number of mitochondria bearing sufficient mutated mtDNA exceed a certain threshold, mitochondrial function becomes impaired and patients manifest clinical symptoms and signs of disease (threshold affect).
During mitosis and meiosis, the proportion of mutant mitochondria in daughter cells can shift, thus changing the genotype and possibly the phenotype (mitotic/meiotic segregation). In addition, mutant mitochondria may utilize the mitochondrial-encoded mRNAs and tRNAs from neighboring normal mitochondria in a process called complementation. Thus, there can be some degree of normal translation of mtDNA-encoded proteins even in mitochondria harboring large DNA deletions.
Different organs have differing susceptibility for mitochondrial abnormalities depending on their energy requirements. Because the central nervous system (CNS) is in constant demand for energy, small decreases in energy production can lead to severe abnormalities. In contrast, skeletal muscle has low energy demands at rest, but these demands drastically increase with exercise. This is the basis for exercise-intolerance in many patients with mitochondrial myopathies.
Primary mutations of mtDNA can only be inherited from the mother. Unlike X-linked disorders that are also passed on only from the mother, women and men are equally affected in inherited mitochondrial diseases, while men are generally more severely affected with an X-linked inheritance pattern. Further, based on the degree of mitochondrial segregation and heteroplasty, all the children of an affected mother may be affected to a variable degree, which is different from autosomal dominant and recessive inheritance patterns.
Mitochondrial disorders are not strictly inherited from an affected mother. Because over 95% of mitochondrial proteins are encoded from nuclear genes, mitochondrial disorders can be inherited in an autosomal dominant [e.g., some forms of progressive external ophthalmoplegia (PEO)], autosomal recessive [e.g., mitochondrial neurogastrointestinal encephalomyopathy (MNGIE) syndrome], and even X-linked (e.g., some forms of Leigh syndrome) fashion. In addition, the presence of mutations involving mtDNA does not imply a maternal/mitochondrial inheritance pattern. In this regard, Kearns–Sayre syndrome (KSS) is associated with large mtDNA deletions, but is sporadic in nature. In addition, as noted earlier there appears to be some nuclear control over the replication and/or maintenance mitochondrial genome. Thus, mutations in some nuclear genes result in syndromes associated with depletion or multiple deletions of mtDNA. These disorders can demonstrate autosomal recessive or dominant inheritance patterns and are caused by defects in enzymes required for mtDNA replication and for maintaining the proper balance within mitochondria of deoxynucleoside triphosphates (dNTPs) that serve as the building blocks for mtDNA (Figs. 30-1,30-3). Replication of mtDNA requires the catalytic subunit of polymerase (encoded by the POLG1 gene), the accessory subunit (encoded by POLG2), and the replicative helicases, twinkle (encoded by PEO1), and DNA replication helicase/nuclease 2 (encoded by DNA2). Mutations in these genes result in mtDNA depletion and/or multiple mtDNA deletions that are found in various mitochondrial disorders [e.g., PEO, MNGIE, sensory ataxia neuropathy dysarthria/dysphagia ophthalmoplegia (SANDO)], which are discussed later.1–6 A delicate balance of the 4 dNTPs (dATP, dGTP, dCTP, and dTTP) are also necessary for mtDNA replication. Several nuclear-encoded enzymes are key in preserving this balance (i.e., TK2, DGUOK SUCLA2, SUCLG1, RRM2B, TYMP, and MPV17). Mutations in these genes also lead to mtDNA depletion and cause various mitochondrial disorders (e.g., mitochondrial depletion myopathy, MNGIE, Navajo neurohepatopathy).1–6 Furthermore, some nuclear genes are responsible for normal mitochondrial dynamics (Figs. 30-1,30-3). For example, fusion of mitochondria is dependent on nuclear-encoded profusion GTPases that are located in the mitochondrial membranes and mitofusin 1 and 2 (MFN1 and MFN2).1–6
Figure 30-3.
Overview of mechanisms underlying the main mitochondrial peripheral neuropathies. (A) MFN2 is located in the outer mitochondrial membrane and interacts with Miro and Milton proteins, which belong to the molecular complex that links mitochondria to kinesin (KHC) motors. (B) MFN2 participates in bringing the outer membranes of two mitochondria into close proximity. Thus, MFN2 mutations can lead to defects in mitochondrial motility along the cytoskeletal microtubular tracks, and to dysfunction in fusion of the outer mitochondrial membranes of opposing mitochondria. Similarly, mutations in OPA1, which is located in the inner mitochondrial membrane, lead to dysfunction in the fusion process of the inner mitochondrial membrane. (C) Loss-of-function mutations in GDAP1, located in the outer mitochondrial membrane, can lead to dysfunction in the mitochondrial fission process, since GDAP1 might be a positive effector of assembly of the fission mediator DRP1. Dysfunction is shown by red oblique bars. (D) Dysfunctions in the respiratory chain can be due to the following: Direct mutations in mitochondrial protein-coding genes (red segment of circular mtDNA) ND1, ND4, and ND6, which encode for subunits of complex I (NADH dehydrogenase), and MTATP6, which encodes for a subunit of complex V (ATP-synthase); mutations in mitochondrial tRNA-coding genes (light blue segment) MTTL1 and MTTK, which lead to dysfunction in transcription of mitochondrial protein-coding genes; or direct mutations in the nuclear gene SURF1 (in green), which encodes an ancillary protein involved in complex IV (cytochrome c oxidase) assembly. Dysfunction in the corresponding respiratory chain complexes are shown by red, light blue, and green asterisks, respectively. Encircled numbers show the number of subunits encoded by mtDNA. (E) Dysfunctions of the respiratory chain can also occur as a result of changes in mtDNA synthesis (red oblique bar), which lead to mtDNA depletion or multiple mtDNA deletions. mtDNA synthesis might be disturbed by the following: Mutations in nuclear genes encoding components of the mitochondrial replisome—that is, polymerase gamma POLG and the helicase C10orf2 genes; loss-of-function mutations (red oblique bar) in genes involved in the synthesis of nucleotides—that is, RRM2B and TYMP—which lead to nucleotide depletion (red lines show inhibition); or mutations in MPV17, a nuclear gene encoding an inner mitochondrial membrane protein of unknown function. Finally, mitochondrial peripheral neuropathies might result from changes in intermediary metabolism that eventually lead to decreased ATP synthesis. Mutations in SLC25A19 inhibit the passage of TPP from the cytosol to the mitochondrial matrix, thus leading to lactate accumulation and an increase in αKG (an intermediate of the Krebs cycle), since TPP is an essential cofactor of PDH and αKGD (green lines show stimulation); similarly, a gain-of-function mutation (green tick mark) in PDK3 might lock PDH in an inactive state, limiting glucose oxidation and favoring a switch toward anaerobic lactate production. mtDNA, mitochondrial DNA; NDPs, nucleoside diphosphates; dNDPs, deoxynucleoside diphosphates; dCTD, deoxycytidine; dCMP, deoxycytosine monophosphate; dCTP, deoxycytosine triphosphate; α-KG, α-ketoglutarate; α-KGD, α-ketoglutarate dehydrogenase; SCA, succinyl-CoA; TPP, thiamine pyrophosphate; PDH, pyruvate dehydrogenase.





There can be significant genetic heterogeneity even within well-defined clinical syndromes. For example, PEO can be associated with multiple mtDNA deletions, point mutations in various mitochondrial tRNA genes, or have no mtDNA mutations. In addition, specific mutations of mitochondrial-encoded genes can manifest with heterogeneous clinical phenotypes. For example, point mutations in the mitochondrial tRNALeu can result in mitochondrial myopathy lactic acidosis and strokes (MELAS), PEO, encephalomyopathy, or a generalized myopathy with exercise intolerance. Variability in clinical phenotype can also be apparent within families with identical mtDNA mutations. The vast clinical and genetic heterogeneity of the various mitochondrial disorders can be explained by the different segregation patterns of mutant mitochondria, the degree of mutant heteroplasty, tissue-specific thresholds, and the severity of the biochemical impairment related to the specific mutations.
Serum creatine kinase (CK), lactic acid, and pyruvate levels can be normal or elevated. In addition, lactic acid levels may also be elevated in cerebrospinal fluid (CSF). Some mitochondrial disorders (e.g., mtDNA depletion) can be associated with renal tubular defects characterized by glycosuria, proteinuria, and aminoaciduria.
Bicycle ergometry can sometimes be a useful test. Low levels of workload lead to an excessive rise in pulse rate and oxygen consumption. The degree of exercise intolerance correlates directly with the severity of impaired muscle oxidative phosphorylation as indicated by the peak capacity for muscle oxygen extraction and mitochondrial mutation load.12,13 The diagnostic value of a constant workload protocol may be superior to an incremental cycle test, but the test is less sensitive for mitochondrial myopathies than simple testing of resting lactate and muscle morphology.14
A forearm exercise test can be performed where bicycle ergometry testing is not available.15 The patient is instructed to open and close their hand (about once every 2 seconds at 40% of maximal voluntary contraction for 3 minutes). A butterfly needle can be placed in the antecubital fossa and venous oxygen and lactate levels can be measured at baseline and each minute during and immediately following exercise. Patients with mitochondrial myopathies and exercise intolerance often demonstrate excessive and prolonged lactate production and paradoxically increased venous oxygen saturation.15 The range of elevated venous PO2 during forearm exercise in mitochondrial myopathy patients (32–82 mm Hg) correlates closely with the severity of oxidative impairment as assessed during cycle exercise.16 Thus, the measurement of venous PO2 during aerobic forearm exercise provides an easily performed screening test that sensitively detects impaired oxygen use and accurately assesses the severity of oxidative impairment in patients with mitochondrial myopathy and exercise intolerance.
Nerve conduction studies (NCS) may be normal or abnormal. Some mitochondrial disorders are associated with a myopathy and/or a neuropathy. The neuropathy can be an axonal [i.e., neuropathy ataxia and retinitis pigmentosa (NARP)] or demyelinating in nature (e.g., MNGIE). Electromyography (EMG) is usually normal, although some myopathies are associated with increased insertional and spontaneous activity as well as early recruitment of small motor unit action potentials (MUAPs), while neurogenic disorders may be associated with decreased recruitment and large MUAPs. Conduction defects may be apparent on electrocardiograms (EKG).
Magnetic resonance imaging (MRI) and CT of the brain as well as electroencephalography (EEG) are typically abnormal in patients with a mitochondrial encephalomyopathy. MRI of skeletal muscle can reveal morphologic changes that resemble muscular dystrophies.17 Magnetic resonance spectroscopy (MRS) with 31P and 1H compounds permits the analysis of ATP, creatine phosphate, inorganic phosphate, and pH in muscle and brain.18,19 In mitochondrial disorders, there is a rapid fall in levels of creatine phosphate and an abnormal accumulation of inorganic phosphates in tissues with exercise. In addition, there is a delay in the recovery of phosphocreatine levels to normal after exercise. These techniques may also be potentially valuable in evaluating efficacy of various treatments.20
If a mitochondrial disorder is suspected from the clinical history and laboratory results, a muscle biopsy may be useful to confirm the diagnosis. Mutational analysis may be done on white blood cells. However, in certain syndromes, this is not as sensitive in finding mitochondrial mutations as in muscle tissue, particularly in those with large mtDNA deletions.
The histopathological abnormalities in nerve biopsies of the various mitochondrial disorders are nonspecific and generally not helpful. However, muscle biopsies are often useful in diagnosing a mitochondrial disorder, particularly if there is significant muscle involvement. The characteristic histological features are the presence of ragged red fibers on the modified-Gomori trichrome stain (Fig. 30-4). An increased number of lipid droplets are also often evident within these abnormal muscle fibers. Oxidative enzyme stains nicotinamide adenine dinucleotide dehydrogenase (NADH), SDH, and cytochrome c oxidase (COX) are also invaluable. The aggregated mitochondria intensely react to NADH and SDH stains forming ragged blue fibers (Fig. 30-4). Some patients with mitochondrial myopathies (in particular disorders not associated with mt-tRNA mutations) may have no ragged red fibers and normal NADH and SDH staining. COX stain (directed against one of the subunits encoded by mtDNA) appears to be the most sensitive stain and can demonstrate scattered muscle fibers with reduced or absent stain (Fig. 30-4). In addition, COX can highlight the subsarcolemmal accumulations of mitochondria. Reduced COX staining can be seen in both ragged red and otherwise normal-appearing muscle fibers. The variability of COX staining in combination with intense SDH staining is characteristic of disorders with mtDNA mutations. Remember, the SDH component of complex II is entirely encoded by nDNA, while 3 of 13 subunits of complex IV (COX) are encoded by mtDNA. Mutations of mtDNA often lead to a proliferation of mitochondria, perhaps in a compensatory response. Because SDH is encoded by nDNA, its transcription is generally increased in disorders caused by mtDNA mutations. The variability of COX staining reflects the heteroplasmic population of mutant and wild-type mitochondria. COX staining is not always abnormal in mitochondrial myopathies. Some patients with MELAS, point mutations in either ND genes or cytochrome b, or multiple mtDNA deletions (e.g., due to POLG1 and other nDNA mutations) can have normal muscle histochemistry, including COX staining.9
Figure 30-4.
Muscle biopsy demonstrates ragged red fibers resulting from the accumulation of abnormal mitochondrial below the sarcolemma of muscle fibers on modified Gomori-trichrome stain (A). Mitochondrial myopathies associated with mtDNA mutations often spare SDH, which is entirely encoded by the nuclear genome. Therefore, muscle fibers with proliferating mitochondria stain intensely with SDH stain—so-called ragged blue fibers (B). The most sensitive stain is for cytochrome c oxidase (COX). Scattered COX-negative fibers are often appreciated in mitochondrial myopathies (C). Combining the COX and SDH stains is very helpful as well. The presence of COX negativity in an SDH-positive fiber (blue staining) is suggestive of an mtDNA mutation, though this may be secondary to mutations in nuclear genes regulating mtDNA (D).




Ultrastructural alterations in mitochondria are usually apparent on EM. These abnormalities include an increased number of normal-appearing mitochondria, enlarged mitochondria with abnormal cristae, and mitochondria with paracrystalline inclusions (Fig. 30-5). The paracrystalline inclusions are accumulations of dimeric mitochondrial creatine kinase (mtCK). This enzyme exists in both a dimeric and octamer form, but the increased radical generation in patients with mitochondrial disorders favors the production and crystallization of dimeric mtCK.21
Mitochondrial enzyme activities can be assayed in muscle biopsy specimens. This can be useful when the routine muscle histochemistry is unrevealing, but the diagnosis of a mitochondrial myopathy is still suspected because of the clinical phenotype. It can also be used to target genes for mutation screening. There is no standard method for performing mitochondrial metabolic analysis. Some centers prefer to assay only fresh muscle biopsy specimens (this is necessary for measurement of substrate oxidation). Rates of flux, substrate oxidation, and ATP production can be measured by polarography or using 14 C-labeled substrates. More commonly, measurement of enzyme activity of each of the individual respiratory complexes is performed on frozen muscle tissue.
Mutation analysis of mtDNA and nuclear genes traditionally have been guided by the clinical phenotype, laboratory features, histochemistry, and biochemistry (Fig. 30-6).1–10 In patients with classic clinical syndromes (e.g., MERRF, MELAS), one can proceed directly toward mutation screening for the most common mutations associated with these disorders. As will be discussed, however, there is wide genetic heterogeneity even within well-defined clinical phenotypes. If a mutation is not found in white blood cells, then a muscle biopsy and mitochondrial enzyme analysis can be performed. Then, screening for mutations can be done based on clinical phenotype aided by histochemical features and biochemical analysis. That said, recent advances in molecular genetics have allowed for less expensive and extensive screening of mtDNA and nDNA by next-generation sequencing and other techniques.
Myoclonic epilepsy and ragged red fibers (MERRF) is characterized by myoclonus, generalized seizures (myoclonic and tonic–clonic), ataxia, dementia, sensorineural hearing loss, optic atrophy, and progressive muscular weakness developing in childhood or adult life.1–3,22–31 The clinical spectrum is variable, which may be a reflection of the percentage of abnormal mitochondria that segregate into the respective tissues. Age of onset, spectrum and severity of involvement, and the course can vary, even within families. Muscle weakness and atrophy can be generalized, but there is a predilection for involvement of proximal arm and leg muscles. In addition, a generalized sensorimotor polyneuropathy and pes cavus deformities may be appreciated. The myoclonus is stimulus sensitive, but can be present at rest. The seizures may be photosensitive. Patients are often misdiagnosed as having juvenile myoclonic epilepsy,32 until other signs or symptoms (e.g., weakness, ataxia) manifest. Unlike KSS and PEO, individuals with MERRF do not usually ptosis, ophthalmoparesis, and pigmentary retinopathy. However, cardiomyopathy with conduction block or heart failure may also be seen in MERRF, particularly those cases presenting early.28 MERRF can also be complicated by ventilatory muscle weakness and associated with life-threatening hypoventilation in the setting of surgery, sedation, or intercurrent infection.28,33 Some patients also manifest with multiple symmetric lipomatosis.34,35
Serum CK can be normal or mildly elevated. Serum lactate can be normal or elevated as well. Generalized slowing of the background activity and bursts of spikes and slow waves may be apparent on EEG. MRI or CT scan of the brain often reveals cerebral and cerebellar atrophy. NCS may demonstrate reduced decreased amplitudes of sensory nerve action potentials consistent with a superimposed axonopathy in some patients.23,36,37 EMG is usually normal, although early recruitment of small MUAPs might be evident in weak muscles.
Muscle histopathology is abnormal as noted previously. Many ragged red fibers and COX-negative fibers are evident as well as fibers with increased SDH staining. Neuronal loss and gliosis of the dentate nuclei, globus pallidus, red nuclei, substantia nigra, inferior olivary nuclei, optic nerves, and cerebellar cortex are apparent on autopsy.24 In addition, demyelination and gliosis are evident in the corticospinal and spinothalamic tracts, and posterior columns.
There is non-Mendelian maternal inheritance of MERRF. Approximately 80% of MERRF causes are caused by a point mutation at nucleotide position 8344 of the mitochondrial genome that results in an A to G transition in the tRNALys gene (MTTK).30,38–40 Of note, there is clinical heterogeneity with this specific mutation as patients can present with PEO, Leigh syndrome, or multiple symmetric lipomatosis.24,35 MERRF has also been described with mutations at other locations in the tRNALys gene (positions 8356 and 8366) and with mutations in the tRNALeu (MTTL1) that is most commonly mutated in MELAS. Other tRNA mutations associated with MERRF include tRNAHis (MTTH), tRNAPhe (MTTF), and tRNASer (MTTS1). In addition, an MERRF clinical phenotype can also be found in patients with multiple mtDNA deletions caused by mutations in the polymerase gamma 1 gene (POLG1) and in ND5 (MTND5). Mutations can be demonstrated by polymerase chain reaction of mtDNA in leukocytes or muscle specimens, but the frequency of abnormal mtDNA is greater in muscle.
As described previously, the mitochondrial tRNA gene mutations impair the translation of mitochondrial-DNA–encoded respiratory chain proteins. Assays of mitochondrial enzyme activity in biopsied muscle tissue reveals diminished activity of complex I and IV. At least 90% of the mitochondria must harbor mutations in order for clinical abnormalities to appear.34
There is no specific therapy for MERRF other than treating the myoclonus (e.g., clonazepam) and the seizures with antiepileptic medications. A slight benefit was reported in a few patients with MERRF treated with creatine monohydrate (5–10 g/day).41,42 Special care must be taken as patients with mitochondrial myopathies can develop marked alveolar hypoventilation in response to sedating medications and anesthetic agents.43,44
MELAS is characterized by muscle weakness, high lactate levels in the serum or CSF, and stroke-like episodes.1–3,44–48 Onset occurs in the first year of life in fewer than 10% with 60–80% developing symptoms and signs of the illness by the age of 15 years.44,47 Rarely, MELAS can present as late as the eighth decade.45 Most affected individuals have recurrent stroke-like episodes manifesting as migraine-type headaches with nausea and vomiting, hemiparesis, hemianopsia, or cortical blindness. These stroke-like attacks may be provoked by exercise or intercurrent infection. Progressive dementia may ensue. Most patients exhibit proximal muscle weakness and complain of easy fatigue and myalgias with exercise.
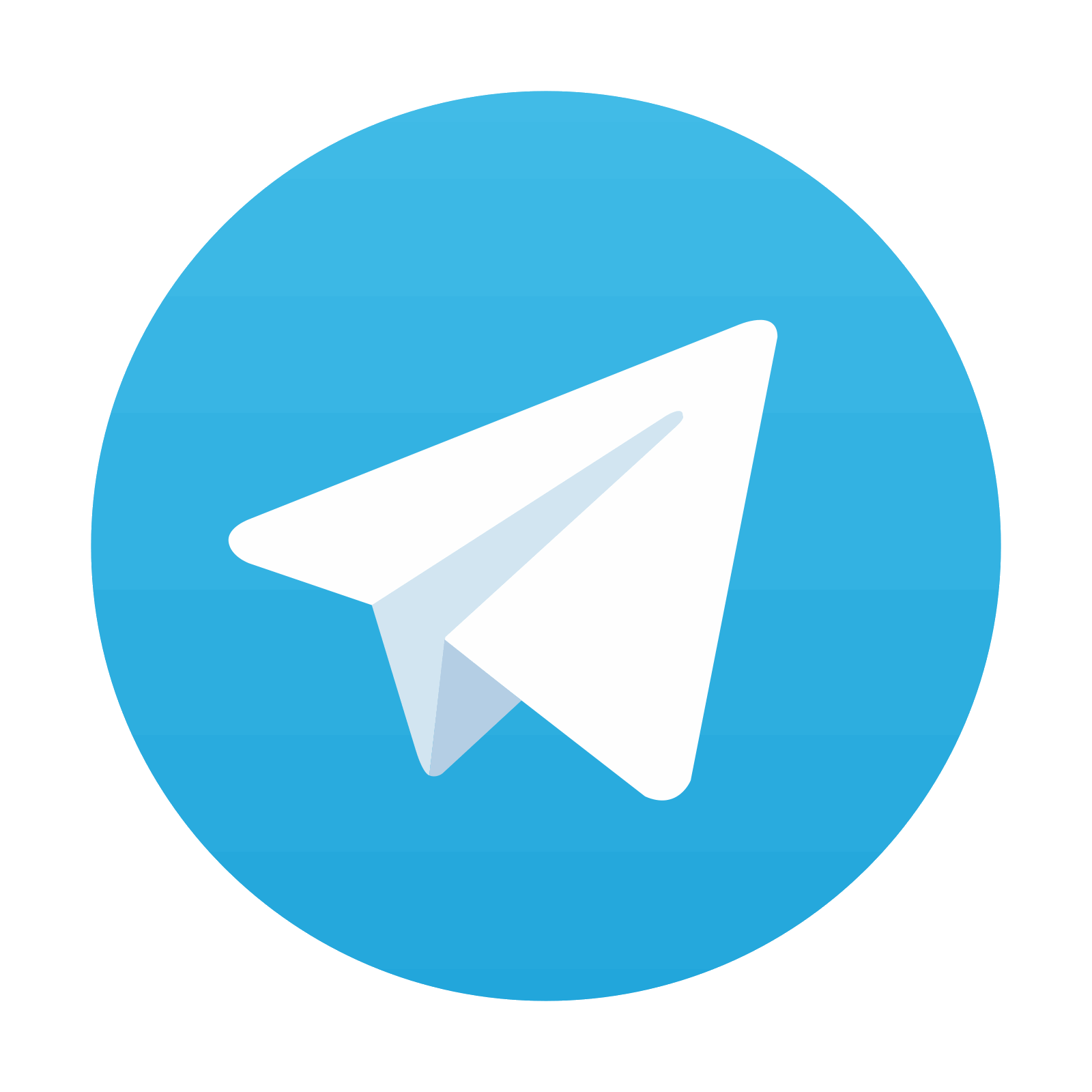
Stay updated, free articles. Join our Telegram channel
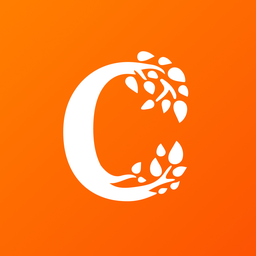
Full access? Get Clinical Tree
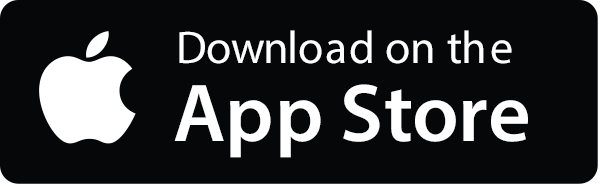
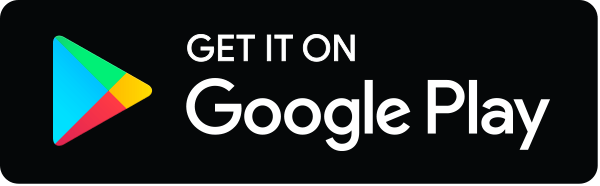