Introduction
This is an exciting time in the field of psychiatry. The advent of rapid-acting antidepressants (ADs) like ketamine has sparked a paradigm shift in how we think about the biological basis of treatment-resistant depression (TRD) and has inspired creative solutions in preclinical model development. The purpose of this chapter is to review current and emerging preclinical research trends capable of characterizing the mechanisms mediating and moderating AD response. As novel approaches continue to emerge, preclinical models will collectively help untangle the heterogeneity inherent within current disease classification systems. In turn, these advances will create new opportunities to more effectively target specific treatments to underlying pathophysiologies using precision medicine approaches currently applied in other fields. Ultimately, the integration of preclinical modeling with translational clinical research is set to transform the way we conceptualize TRD. However, bridging this gap will require refinement of current preclinical research strategies and the establishment of interdisciplinary collaborations across psychiatry, neuroscience, bioinformatics, molecular pharmacology, and other fields.
Common mechanisms of antidepressant response in depression
First-line ADs work primarily by blocking monoamine reuptake mechanisms, which almost instantly elevate monoamine neurotransmitter levels at the synapse. Yet, for a long time, there remained an apparent disconnect between the presumed neurobiological mechanisms of these ADs (overcoming a deficit in monoamine neurotransmitter levels) and time to clinical AD response (several weeks). Mark Hyman and Eric Nestler proposed a model of initiation and adaptation in 1996 ( ) to help explain this gap, suggesting that AD responses emerged via indirect pathways dependent on the initiation of molecular signaling cascades that, in turn, coordinate cellular neurotrophic adaptations over weeks to months ( Fig. 5.1 ).

Synaptic dysfunction in depression
Preclinical models have demonstrated that AD mechanisms work on a backdrop of synaptic dysfunction induced through various exposures, including chronic stress and/or inflammation, and accelerated by underlying genetic vulnerabilities ( ). Neurons and glia make up the majority of cells in the brain and form complex neural circuits. Effective communication within these circuits requires highly integrated electrochemical signaling, which depends on efficient and effective synaptic communication. At the synapse, stored neurotransmitters are released and bind to receptors on pre- and postsynaptic membranes to trigger intracellular changes and ongoing propagation of the electrochemical signal throughout the circuit. The brain’s highly adaptive nature enables active synapses to become strengthened, thereby increasing the efficiency of signal transduction along this same pathway in the future, while inactive synapses are pruned, effectively whittling away unused connections. In depression, this process is maladaptive, with excessive synaptic pruning occurring in specific brain regions such as the prefrontal cortex (PFC) and hippocampus ( ; ). This disrupts activity within the mesocorticolimbic mood regulatory circuit to impact a range of essential functions, including motivation, perception, cognition, and emotion regulation ( ; ). Recent work suggests that both long-term activity-dependent changes in synaptic strength (synaptic plasticity) and shifting set points for these that result in relatively “hard-wired” changes that persist over the longer term (metaplasticity) and are essential for establishing or reversing neurobiological adaptations contributing to depression ( Fig. 5.1 ).
Synapses are situated on dendritic spines, which are dynamic structures that functionally serve as the locus of long-term synaptic plasticity ( ). At the tip of most dendritic spines, there is a dense region that harbors glutamate receptors and other protein complexes, namely a postsynaptic density (PSD), or postsynaptic synapse ( ). The size of a dendritic spine is proportional to the size of its PSD, the number of glutamate receptors, and, in turn, the synaptic strength or capacity for synaptic communication. For example, the volume of the spine-head is directly proportional to the number of postsynaptic receptors ( ), and the presynaptic number of docked vesicles ( ). Synaptic plasticity modifies information flow throughout the circuit and the consequent cognitive and behavioral outputs. Prolonged exposure to stress and inflammation dynamically regulate dendritic complexity, particularly within the PFC and hippocampus, regions that play an important role in the depression circuit ( ; ; ). The loss of synaptic connectivity in these critical nodes of the mood circuit profoundly impacts mood and cognitive function. This inability to maintain synaptic integrity and plasticity in depression represents a failure of one of the most fundamental and critical functions of the brain, namely the ability to communicate and store information and make appropriate, adaptive, and efficient responses to subsequent inputs ( ). While this critical brain function has been best studied for its role in learning and memory, it is now clear that it also plays a crucial role in the pathophysiology of depression and serves as the underlying mechanism of AD action ( ; ; ; ). Failure to effectively engage this mechanism is now thought to underlie the poor clinical response profiles observed in TRD ( ). The reasons for this target engagement failure are likely many and varied. Preclinical models will be critical for both the discovery of these rate-limiting mechanisms and their translation into innovative biomarker and treatment strategies for the clinic.
Antidepressant regulation of synaptic plasticity
Antidepressant treatments elicit region-specific structural changes via the induction of dendritic arborization, spinogenesis, improved cell survival, enhanced hippocampal neurogenesis, angiogenesis, and gliogenesis, a dynamic process known as synaptic plasticity ( ; ). Synaptic plasticity is an essential neurobiological mechanism that plays a vital role in brain development, maturation, and ongoing life-long learning processes and is implicated in several major neuropsychiatric and neurodegenerative disorders ( ; ; ). Synaptic plasticity occurs in part through activity-dependent, long-lasting modifications of synaptic strength, more commonly referred to as long-term potentiation (LTP) and long-term depression (LTD). The strengthening of synapses through LTP is triggered by N -methyl- d -aspartate (NMDA) receptors (NMDARs). A large NMDAR-dependent increase in dendritic spine calcium concentrations leads to activation of intracellular signaling cascades involving several protein kinases, most importantly Ca 2 + /calmodulin-dependent protein kinase (CaMK). This leads to increased single-channel conductance of synaptic α-amino-3-hydroxy-5-methyl-4-isoxazole propionic acid receptor (AMPARs) and synaptic proteins such as PSD-95. In parallel, structural changes within the synapse occur, such that the size of the PSD and dendritic spine are increased, leading to an increase in synaptic strength, maintenance and ultimately impacting synaptic plasticity ( Fig. 5.2 ).

Classical ADs elicit plasticity via three main routes: receptor blockade, reuptake inhibition, and inhibition of enzymes that degrade monoamines, particularly monoamine oxidase. The resulting excess of monoamine neurotransmitter availability at the synapse results in the regulation of postsynaptic G protein-coupled receptors (metabotropic receptors), which couple to a variety of second messenger systems, including the cAMP-protein kinase A (PKA)-cAMP response element-binding (CREB) pathway ( ). These actions result in slow adaptive processes that impact gene transcription and brain-derived neurotrophic growth factor (BDNF) signaling to ultimately alter synaptic regulatory elements that work to restore monoamine balance at the synapse ( ; ; ; ; ).
In contrast, rapid-acting ADs such as ketamine, which functions as an NMDAR antagonist, enable synaptic plasticity via more direct activation of neurotrophic mechanisms ( ). A distinct difference between ketamine and slower-acting classical ADs is that ketamine triggers a rapid (within hours) upregulation of growth factor signaling and dendritic spine development in the PFC ( ; ). Studies have provided evidence of ketamine activation of AMPAR, an ionotropic transmembrane glutamate receptor that mediates fast synaptic transmission and is essential for the generation of LTP. The activation of AMPAR leads to the release of BDNF and downstream activation of growth and metabolic signaling cascades, such as the phosphoinositide 3-kinase (PI3K/Akt/mTOR) signaling pathway and the MAPK/ERK signaling pathway in the limbic system to mediate AD effects ( ). The downstream actions of AMPA receptor activation lead to an increase in neurotrophins such as brain-derived neurotrophic factor (BDNF) levels and activation of its receptor tropomyosin receptor kinase B (TrkB) ( Fig. 5.2 ).
Neurogenesis
Antidepressants stimulate the generation of newborn neurons from neural stem cells in the adult hippocampus, a process known as neurogenesis ( ; ), which takes about 4 weeks to form synaptic connections ( ) and contributes to antidepressant-like effects in rodents ( ; ; ). In mammals, this process is limited to select regions, the best-studied of which is the subgranular layer of the dentate gyrus of the hippocampus. Herein, adult newborn neurons are formed from quiescent neural progenitor stem cells, which differentiate into mature granule cells, which become integrated into the existing hippocampal circuitry. It is becoming increasingly clear that neurogenesis in the hippocampus and other regions plays a key role in both the pathophysiology and treatment of mood disorders ( ; ). There is a significant reduction in hippocampal volume in depressed relative to healthy subjects ( ; ), with contrasting increases in volume in other regions, including the amygdala ( ). Such structural differences are confirmed in postmortem studies, demonstrating, for example, more significant reductions in hippocampal histological sections from depressed patients relative to control subjects ( ).
Hippocampal overexpression of neurotrophic signaling factors BDNF or CREB mimics both the structural and behavioral effects of sustained antidepressant treatment ( ). In line with this, studies have shown that antidepressant drugs, including tricyclic antidepressants and selective serotonin reuptake inhibitors, increase neuronal proliferation in the dentate gyrus when administered chronically ( ). However, the rate of cell proliferation and programmed cell death in the dentate gyrus of mice chronically treated with antidepressants does not appear to be dependent on BDNF. However, activation of the BDNF receptor TrkB is essential for the long-term survival of newborn neurons ( ). These effects are dependent on chronic administration of antidepressant therapy, consistent with the time course of antidepressant treatments ( ). This suggests that the regulations of BDNF and TrkB are involved in antidepressant treatment response and that this is directly reversing a critical pathophysiological feature of the illness. Whether this is a common mechanism of AD response remains to be determined.
Studying antidepressant response in preclinical models
Significant advancements have been made toward our understanding of the fundamental neurobiological mechanisms mediating AD response over the last decade, on the back of discovering the rapid-acting AD potential of ketamine. While this has made clear the critical role that neurotrophic mechanisms play in this process, the factors that interfere with this to effectively limit AD response are not well understood. Suitable preclinical scientific models are needed to address this gap. Development and use of depression models is challenging due to the complex nature of the disorder, and much has been written on their limitations ( ; ; ). No one model can represent the full complexity of the human condition, particularly TRD. Arguably, to hold such aspirations is somewhat misplaced, and preclinical approaches that focus instead on the biological mechanisms of AD response and nonresponse could more effectively inform on the mechanisms contributing to treatment resistance. In this way, each model must be within the context of its underlying strength as part of a greater whole, referring back to the human condition for verification and optimization ( ). The factors that can interfere with AD response are many and varied, and include biological factors such as genetic ( ; ), inflammation ( ; ; ; ), neurotrophins ( ; ; ; ), gut-brain interactions ( ; ), neuroendocrine dysregulation (i.e., glucocorticoid resistance) ( ), and bioenergetic processes ( ; ). Consequently, the development of useful preclinical tools to investigate AD mechanisms must take these into account, collectively contributing to the development of a multi-scale, systems biology approach to addressing treatment resistance in depression ( ). However, as for the human condition, there will be heterogeneity in the presence and impact of these. Thus a more direct focus on how these factors impact neurotrophic responses to ADs may enable us to more effectively screen for these treatment response interrupters in a manner that can enable personalized treatment options, potentially in combination with other clinical tools or recommendations that remove or reduce biological barriers to plasticity, and in turn AD response.
Animal models
Our understanding of the specific neurobiological mechanisms contributing to AD response within the context of animal models of depression positions us optimally to determine which factors functionally limit this molecular machinery’s effective engagement. While never effectively recapitulating the human condition of depression, animal models offer the unique capacity to dissect out factors capable of altering the biological efficacy of ADs ( ; ). When used in partnership with clinical paradigms, they add significant value to the scientific endeavor to understand and more effectively treat what we currently, and somewhat pessimistically, define as TRD.
Advantages of research with animal model systems include:
- 1.
high degree of experimental control over the environment;
- 2.
ability to monitor biological effects across central and peripheral compartments in a highly selective, cell-specific manner;
- 3.
focus on pathways of communication between central and peripheral compartments (including nervous and immune systems); and
- 4.
assessment of integrated neurobiological and biobehavioral processes.
Without a doubt, experimental animal models are an indispensable preclinical research tool. To effectively model human depression, they are required to have face (phenotypic similarities), construct (neurobiological similarities), and predictive (treatment response similarities) validity ( ). The latter requirement has particularly limited our capacity to develop valid models of TRD. Rodent models have long used different pathogenic factors to elicit depression-like behaviors ( Table 5.1 ), including stress ( ; ), genetic mechanisms ( ; ; ; ), inflammation ( ; ; ), aging ( ; ), and targeted network disruption ( ). These models have also been used to study the biological basis of AD responses, including AD treatment resistance ( ; ; ). These rodent models are used to determine how pathogenic mechanisms (e.g., chronic stress exposure) affect the brain to impact behavioral responses. In contrast, behavioral tests, or screening models, are used to access specific behavioral features that can be changed due to treatments. There is increasing attention being placed on improving our current psychiatric and translational paradigms to model human conditions more effectively ( ).
Models | Depressive-like behavior | Biological alterations | Antidepressant response |
---|---|---|---|
Wistar Kyoto rats ( ) | Increased learned helplessness; increased immobility in the forced swim test; decreased activity in the open field test | Dysregulation of the hypothalamic-pituitary-adrenal (HPA) and hypothalamic-pituitary-thyroid (HPT) axes | Resistant to fluoxetine. Responsive to ketamine |
Congenital learned helplessness (cLH) rats ( ) | Deficient reward perception, depressive-like cognitive bias, increased LH and anhedonic; respond less to sucrose and show hyperactivity during open field test | Increased activity in the ACC and habenula; higher glutamate/GABA ratios in the hippocampus and prefrontal cortex | Responsive to deep brain stimulation |
High anxiety behavior (HAB) mice ( ) | Increased immobility in the forced swim test | Reduced hippocampal neurogenesis | Response to chronic noradrenergic drugs (reboxetine or desipramine), but resistant to chronic selective serotonin reuptake inhibitors (SSRIs) including fluoxetine, paroxetine and citalopram. Good response to deep brain stimulation |
5-HT1a mice ( ) | Increased anxiety and response to stress | Physiological responses to stress | No response to chronic SSRIs |
5-HT1b mice ( ) | Increased locomotor response to stress | Stress-induced locomotor responses | Response to SSRIs |
Unpredictable chronic mild stress (UCMS) mice ( ) | Impairment of rewarded behavior | Hypothalamic pituitary adrenal dysregulation, brain derived neurotrophic factor (BDNF) alterations, reduced monoamine levels | Response to antidepressants except when on a high-fat diet when they display resistance to fluoxetine. Good response to ketamine |
Cannabinoid receptor type 1 knockout (CB1 KO) mice ( ) | Anhedonia, increased response to chronic mild stress | Impaired cannabinoid receptor type 1 affecting mesoaccumbens dopamine signaling and plasticity | Reduced response to desipramine or paroxetine subchronic administration |
Organic Cation Transporter 2 (OCT2) null mutant mice ( ) | Increased anxiety, increased response to chronic mild stress and immobility in the forced swim test | Decreased levels of serotonin and noradrenaline | Response to noradrenaline and serotonin ADs, but resistance to venlafaxine |
Dopamine β-hydroxylase (DBH) deficient mice ( ) | Increased response to stress | Lack of norepinephrine and epinephrine; low heart rate, hypotensive, attenuated circadian blood pressure rhythm | Resistance to acute administration of desipramine, reboxetine pargyline, bupropion and the SSRIs fluoxetine, sertraline and paroxetine. Response to citalopram |
Hypothalamic pituitary adrenal (HPA) axis disruption ( ) | Anhedonia | Increased levels of serotonin, norepinephrine and epinephrine following stress; low dopamine | Resistance to tricyclic antidepressants, reversed with lithium augmentation. Bupropion response and partial ketamine response observed. Responsive to deep brain stimulation |
Rodent animal models
Rodent models are powerful tools for investigating the mechanistic role of biological factors associated with AD resistance in clinical and epidemiological studies. Early animal research in depression aimed to elucidate psychological processes influencing vulnerability to depression-like behavior. Building on this, more recently, animal models have been used to address neurobiological mechanisms regulating behavioral states of relevance to depression. These rodent models of “depression” have historically been validated via antidepressant reversal of “depression-like” behaviors, limiting their potential for use in the study of TRD. Alternative approaches are now needed to explore the mechanisms governing antidepressant nonresponse ( ). This critical next step builds logically on the now well-established mechanisms of antidepressant response, allowing for the discovery of the pathophysiological processes contributing to poor antidepressant outcomes.
Rodent models of TRD, as for depression more broadly, involve a specific manipulation (a procedure used to elicit abnormal behavior) and an experimental measure (a behavioral output, often associated with biomarkers, used to measure AD response; Fig. 5.3 ).

Rodent behavioral tests of AD response
Progress toward modeling AD resistance and studying potential novel therapies therein requires a paradigm shift away from stress-based models of depression-like behaviors ( ), with a new focus on screens of AD response and nonresponse ( ). Indeed, it makes intuitive sense that the ideal models to investigate the potential induction and/or reversal of factors contributing to TRD will be precisely those models in which conventional antidepressants are ineffective. For decades behavioral screening tests for ADs have been used primarily for the development of new treatments. Some of the procedures that are most frequently used in AD research, such as the forced swim test (FST) ( ) and the tail suspension test (TST) ( ), involve acute or chronic treatment administration and provide effective first pass screens of AD effects on active versus passive behavioral coping responses to inescapable stress. An AD-induced shift toward increased active stress coping (climbing or swimming in the FST or climbing in the TST) serves as a proxy behavioral readout for AD effects on key regions of the neural network, including the infralimbic and prelimbic PFC, as well as the mesoaccumbens dopamine systems, the stimulation of which alone is sufficient to enhance active coping behaviors.
Even when administered acutely, drug effects in this paradigm are considered predictive of longer-term AD efficacy in human patients. When used to test novel compounds, these behavioral screens can be undertaken in nondepressed animals. Thus, the FST behavioral assay is considered a useful, predictive screening tool for antidepressant-like activity, rather than an animal model of depression per se ( ). When misrepresented as the latter, these tests rightly draw intense criticism. However, their utility for rapid AD screens should not be hastily dismissed. Indeed, the ability to demonstrate efficacy to specific AD treatments at baseline and then block this through induction of AD resistance enables direct ascertainment of the neurobiological mechanisms implicated in AD nonresponse, which may have physiologic relevance for TRD. Such models have demonstrated utility in the search for mechanisms blocking AD efficacy and uncovering related biomarkers of response. Within this context, rapid behavioral screens of AD response can help discover mechanisms moderating AD nonresponse of relevance to clinical TRD.
Translational rodent behavioral tests
Moving from predictive AD screens to translational behavioral models of TRD is essential for understanding the core pathophysiological underpinnings of distinct behavioral phenotypic features. Such approaches have been informed by the United States National Institute of Mental Health Research Domain Criteria (RDoC) framework, which aims, in part, to develop research paradigms with broad trans-species applications ( ). In so doing, this approach aims to integrate biological, behavioral, and clinical data to explain transdiagnostic dimensions of human functioning that span the full behavioral spectrum. Importantly, through the development of behavioral tests that can be used across several species, from rodents to humans, this approach allows for discovery of neurobiological underpinnings of specific behavioral features (e.g., distinct reward processes dependent on specific neurobiological mechanisms ( ). In the context of preclinical models of TRD, this will help establish a more complete picture of how discrete clinical presentations can impact AD treatment response, potentially allowing for the development of a set of diagnostic screening tools for treatment selection. In this way, by simulating essentially the same process across species, the behavioral models share necessary homology with specific aspects of psychiatric disorders and provide significant translational value ( Table 5.2 ).
Behavioral test | Measurement | Validity | Value |
---|---|---|---|
|
|
|
|
|
|
| |
|
| ||
|
| ||
| Exert effort to escape or passive coping |
|
|
|
| ||
|
| ||
Effort-related choice task | Choice to exert effort for more salient reward |
|
|
|
| ||
|
| ||
| |||
Novelty suppressed feeding | Motivation to seek reward relative to the anxiety induced by the challenge |
|
|
|
| ||
|
| ||
| |||
| |||
Elevated plus maze (or similar) | Anxiety-related avoidance behavior |
|
|
Light/dark box |
|
| |
| |||
|
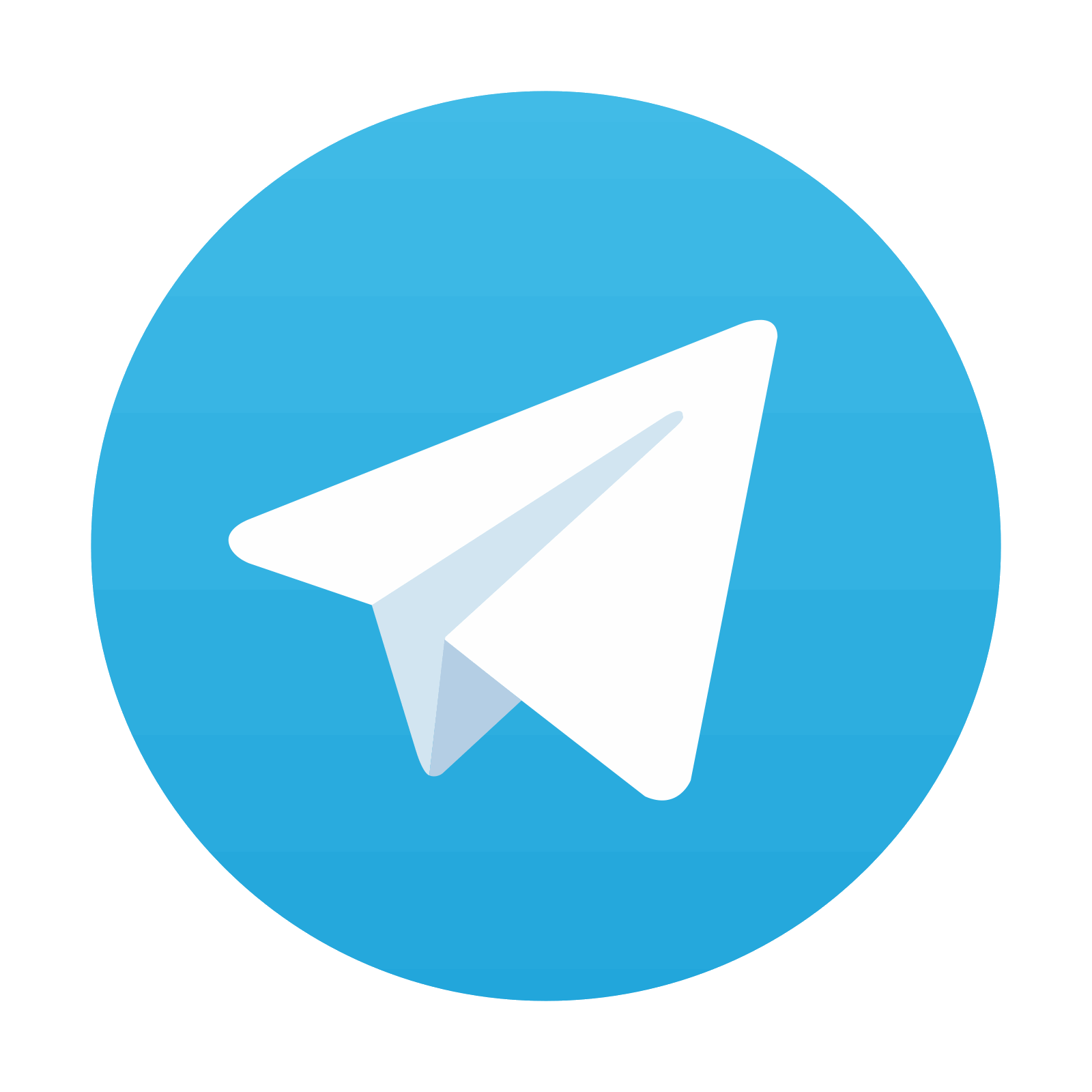
Stay updated, free articles. Join our Telegram channel
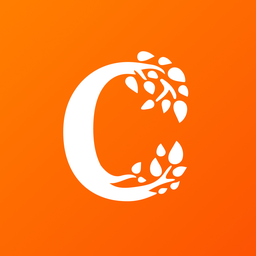
Full access? Get Clinical Tree
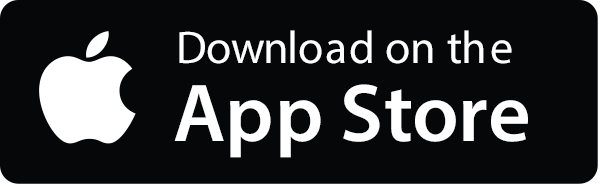
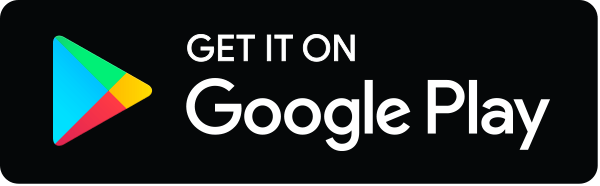
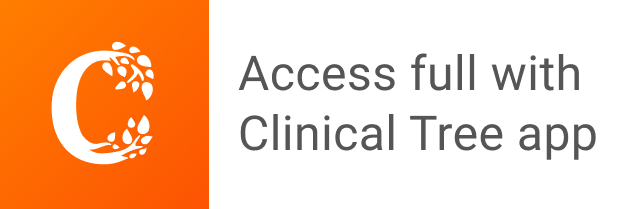