div class=”ChapterContextInformation”>
9. Animals Models for Trigeminal Autonomic Cephalalgias
Keywords
Cluster headacheTrigeminovascularAutonomicParasympatheticTrigeminal autonomic reflexAnimal model9.1 Introduction
- 1.
Severe or very severe, unilateral, trigeminal distribution of pain, sometimes described as the worst pain experienced by humans [2]
- 2.
Lateralised-associated symptoms, including cranial autonomic features [3], such as lacrimation, conjunctival injection, and nasal congestion, and a local third-order sympathetic lesion due to carotid swelling [4]
- 3.
Cluster headache | Paroxysmal hemicrania/CPH | SUNCT/SUNA | |
---|---|---|---|
Sex F:M | 1:3 | 1:1/7:1 | 1:1.2 |
Prevalence | 0.1% | 1/50,000 | 1/15,000 |
Pain type Severity Site | Stabbing, boring Excruciating Orbit, temple | Throbbing, boring, stabbing Excruciating Orbit, temple | Burning, stabbing, sharp Severe to excruciating Periorbital |
Attack frequency | 1/alternate days to 8/day | 1–40/day (>5/day most of the time) | 3–200/day |
Duration of attack | 15–180 min | 2–30 min | 5–240 s |
Autonomic features | Yes | Yes | Yes (mainly conjunctival injection and lacrimation –SUNCT) |
Abortive treatments | Sumatriptan, oxygen | None | None |
Preventive treatments | Verapamil, methysergide, lithium | Indomethacin (absolute response) | Lamotrigine, topiramate, gabapentin |
9.2 Animal Models: Putative Mechanisms
Generally, the development of animal models for a particular disorder has two major aims: to help understand its pathophysiology and to aid in the identification, development and screening of novel and effective therapeutic targets. It is therefore important that these models clearly translate the clinical features of a disorder, using what is currently known about its pathophysiology. These include the anatomy, physiology, pharmacology, molecular biology and genetics, as well as response to treatments. Translating all of these components into a single preclinical approach is often complex, so that only one or two may be captured in a single model. However, the likelihood is that the more features that are translated, the more accurate and reliable the animal approach. In the case of TACs, the very clear classification of symptoms for diagnosis [1] and the well-defined clinical features represent a huge advantage when trying to develop a translational preclinical approach. However, with that being said, historically, there has been a dearth of animal models for TACs. The majority of our understanding comes from clinical research and studies in patients experiencing headache attacks, as well as preclinical studies into generalised primary headache mechanisms, where the anatomy and physiology of the trigeminovascular and cranial autonomic systems are likely shared across many headache disorders.
9.2.1 Evidence from Clinical Studies
A defining feature of TACs compared to other primary headaches is a very clear and reproducible activation in the posterior hypothalamic grey matter, demonstrated in cluster headache [14], paroxysmal hemicrania [15], SUNCT [16] and hemicrania continua [17]. Furthermore, deep brain stimulation of this region has been shown to relieve symptoms in cluster headache [18], chronic paroxysmal hemicrania [19] and SUNCT [20, 21]. In an experimental clinical approach for TACs, capsaicin injection into the forehead produces pain, and many of the vascular changes present during TACs, but no hypothalamic (or even brainstem) activation [22]. The implication is that the trigeminally mediated pain and vascular changes are not the cause of the hypothalamic activation; rather hypothalamic activation causes the subsequent activation of trigeminovascular and cranial autonomic pathways, resulting in TAC symptoms. There is also evidence of release of calcitonin gene-related peptide (CGRP) and vasoactive intestinal peptide (VIP) into the extracranial vasculature during attacks of cluster headache [23] and of chronic paroxysmal hemicrania [24], and release of pituitary adenylate cyclase-activating peptide (PACAP) during cluster headache attacks [25]. The different TACs also have very specific response to treatments (Table 9.1), which can be used as a tool in developing an animal model of TACs. Indeed, CGRP release during cluster headache attacks is normalised by treatment with oxygen or sumatriptan, but not by opioids [23], indicative of the important role of this peptide in cluster headache pathophysiology.
9.2.2 Evidence from Preclinical Studies

Anatomy and pathophysiology of trigeminal autonomic cephalalgias (TACs). The trigeminal distribution of pain in TACs is likely mediated by activation of the trigeminovascular system. This includes the nociceptive-specific nerve fibres that originate in the trigeminal ganglion (TG) and innervate the peripheral cranial vasculature and its central projection to the trigeminal nucleus caudalis (TNC) and its extension to the cervical spinal cord (the trigeminocervical complex; TCC). Nociceptive incoming signals to the TCC ascend to higher brain structures (purple projections) including the midbrain and specific hypothalamic nuclei; the posterior (PH), supraoptic (SON) [40], ventromedial (VMH) and paraventricular hypothalamic nuclei (PVN) [41]; as well as thalamocortical neurons. Dural nociceptive activation also causes neuronal activation in the superior salivatory nucleus (SuS) within the pons [33], through a trigeminal autonomic reflex arc (grey neuron), which is the origin of cells of the autonomic parasympathetic projection to the cranial vasculature [35]. This efferent projection is predominantly through the greater petrosal nerve (green neuron), a branch of the facial (VIIth) cranial nerve (sky blue neuron), and its relay with the sphenopalatine ganglion (SPG). Cranial autonomic symptoms in TACs are believed to result from activation of this trigeminal autonomic reflex arc to the SuS and its projection to the cranial vessels and lacrimal glands. Descending projections (red and yellow neurons) from PH [70], PVN, lateral (LH) [34], dorsomedial (DMH) and preoptic (PON) hypothalamic nuclei to the TCC (red projections), SuS [34–37] and the sympathetic nervous system (both yellow projections) are thought to modulate and control both trigeminovascular nociceptive transmission (purple network of neurons) and parasympathetic (green)/sympathetic (orange) autonomic projections to the cranial vasculature that result indirectly or directly, respectively, in cranial autonomic symptoms ipsilateral to head pain. A third-order sympathetic nerve lesion (orange projection), in part mediated by internal carotid artery (ICA) vasodilation, is thought to result in Horner’s syndrome. AH anterior hypothalamic area, DH dorsal hypothalamus, MB mammillary body
9.3 Animal Models of TACs
The development of an animal model for a disorder requires that it translate at least one aspect of the clinical phenotype, mediated by the same pathophysiology as described in humans. However, the presence of more than one feature would likely improve its translation and reliability. Furthermore, to validate the approach, it would also require a similar response to treatments. With respect to TACs, this includes the combination of lateralisation of trigeminal distribution of pain, and associated cranial autonomic features, and some degree of cyclical recurrence of attacks, likely mediated by hypothalamic manipulation. It should also respond to treatments of proved efficacy in TACs, such as inhaled oxygen and sumatriptan for cluster headache, indomethacin for paroxysmal hemicrania and topiramate, lamotrigine, or gabapentin for SUNCT/SUNA.
9.3.1 Trigeminal Distribution of Pain
9.3.1.1 Dural Nociceptive Activation
The trigeminal distribution of pain is perhaps the most studied in primary headaches, and while the development of many assays was predominantly to study migraine pathophysiology and screen therapeutics, there is considerable overlap for them to be relevant to other primary headaches. Based on the clinical studies of dural vasculature manipulation and pain referral in orbital, peri-orbital or temporal region [26, 27], dural nociceptive activation, driven by electrical stimulation or chemical mediators, is thought to trigger trigeminovascular nociceptive afferents that are activated during headache. This produces dural vasodilation [38] and neuronal activation in the trigeminocervical complex [28, 39], the SuS [33] and higher pain processing structures, including various hypothalamic nuclei [40, 41], as well as midbrain structures [33, 42]. Dural electrical stimulation also causes an increase in levels of CGRP, VIP and PACAP within the extracranial vasculature [43, 44], similar to findings in TACs.
A limitation of this assay is that it perhaps generically maps the neurophysiological changes found across many primary headache disorders that do not specifically relate to TACs. As an example, imaging studies during TACs do not demonstrate midbrain activation, more commonly associated with migraine pathophysiology, yet here we see activation within many structures unrelated to TACs. Also, its response to treatments does not fully match that of TACs. 100% oxygen treatment is known to specifically relieve symptoms of cluster headache, and lamotrigine is used as a preventive for SUNCT/SUNA. Whereas oxygen is unable to inhibit neurogenically mediated dural vasodilation or neuronal activation in the trigeminocervical complex [45], lamotrigine similarly has no effect on dural-nociceptive neuronal responses [46]. In fact only drugs that are effective in treating both migraine and TACs, such as triptans [47–49], non-steroidal anti-inflammatory drugs (NSAIDs) [50, 51] and topiramate [52, 53] are effective in this assay. While nociceptive trigeminovascular activation is very relevant to the pathophysiology of TACs, this model perhaps lends itself more to understanding migraine and screening for migraine therapeutics, rather than TACs. A further limitation is that without a measure of autonomic symptoms, it is difficult to translate this animal model to wider aspects of TAC pathophysiology.
9.3.1.2 Nitrergic Activation of Trigeminovascular Pain Pathways
Nitric oxide (NO) donors, such as nitroglycerin (NTG), are known to provoke cluster headache in patients [54–56] and cause the release of CGRP from the extracerebral vasculature during an attack phase [55]. NTG-provoked cluster headache also physiologically resembles spontaneous cluster headache with craniovascular vasodilation and neuronal activation in the hypothalamus [57]. In preclinical studies, NO donors cause craniovascular vasodilation [58] and activation and sensitization of primary afferent and second-order trigeminovascular neurons [59–62], with increased immunoreactivity for CGRP in the trigeminal ganglion [63] and depletion of CGRP stores in the TNC [64]. Furthermore, some of these nitrergic responses are inhibited by triptans [58], NSAIDs, specifically indomethacin [58, 65], topiramate [52] and also CGRP receptor antagonists [59, 66]. A clear disadvantage to this assay is that when cluster headache patients are in remission, NTG does not trigger a cluster attack or cause the release of CGRP or produce hypothalamic activation [14, 55, 57]. Also, it is assumed that animals are in a ‘naïve’ state and thus not suffering from cluster headache or another TAC. NTG is also known to trigger migraine [67] and TTH [68] in sufferers of these primary headache types, and the NTG-mediated craniovascular and trigeminovascular neuronal changes are not specific to TACs. It seems NTG in animal models is useful in helping to understand the neurovascular neuronal changes that take place in primary headaches, but it is more difficult to generalise these changes to one specific primary headache. More clues could be derived by the study of the different temporal delay of NTG-induced headache response in cluster headache and migraineurs. The latency of onset of the spontaneous-like attacks is indeed significantly shorter in cluster headache patients [55, 56], as compared with migraine subjects, which suggests possible different activation of pathways/intermediaries.
9.3.2 Trigeminal Distribution of Pain with Cranial Autonomic Features
9.3.2.1 Oral or Nasal Capsaicin Injection
It is perhaps more relevant to demonstrate more than one symptom in a preclinical approach to TACs, such as trigeminal distribution of pain and cranial autonomic symptoms. Similar to the capsaicin studies in patients, one approach has used oral and intranasal capsaicin in rodents [69]. This caused blood flow changes in dural arteries as a measure of trigeminovascular activation, and lacrimation, measured by placing filter paper to the medial angle of the eye and the change in weight used as an indicator of activation of the autonomic pathway. These responses were reversed by hexamethonium bromide, an autonomic ganglion blocker. The implication is that oral or intranasal capsaicin causes activation of the trigeminal-autonomic reflex to produce cranial vascular changes and lacrimation. While this model demonstrates several symptoms of TACs, there are reservations as to its clinical relevance. In clinical studies, the capsaicin model does not produce the signature hypothalamic activation of TACs but only the craniovascular changes [57]. Also, this approach has not been validated with TAC-specific treatments, such as oxygen or indomethacin. Perhaps this approach is a good example of trigeminal-autonomic activation, but lacking specific hypothalamic activation, it does not fully translate to the pathophysiology of TACs.
9.3.2.2 Superior Salivatory Nucleus Stimulation

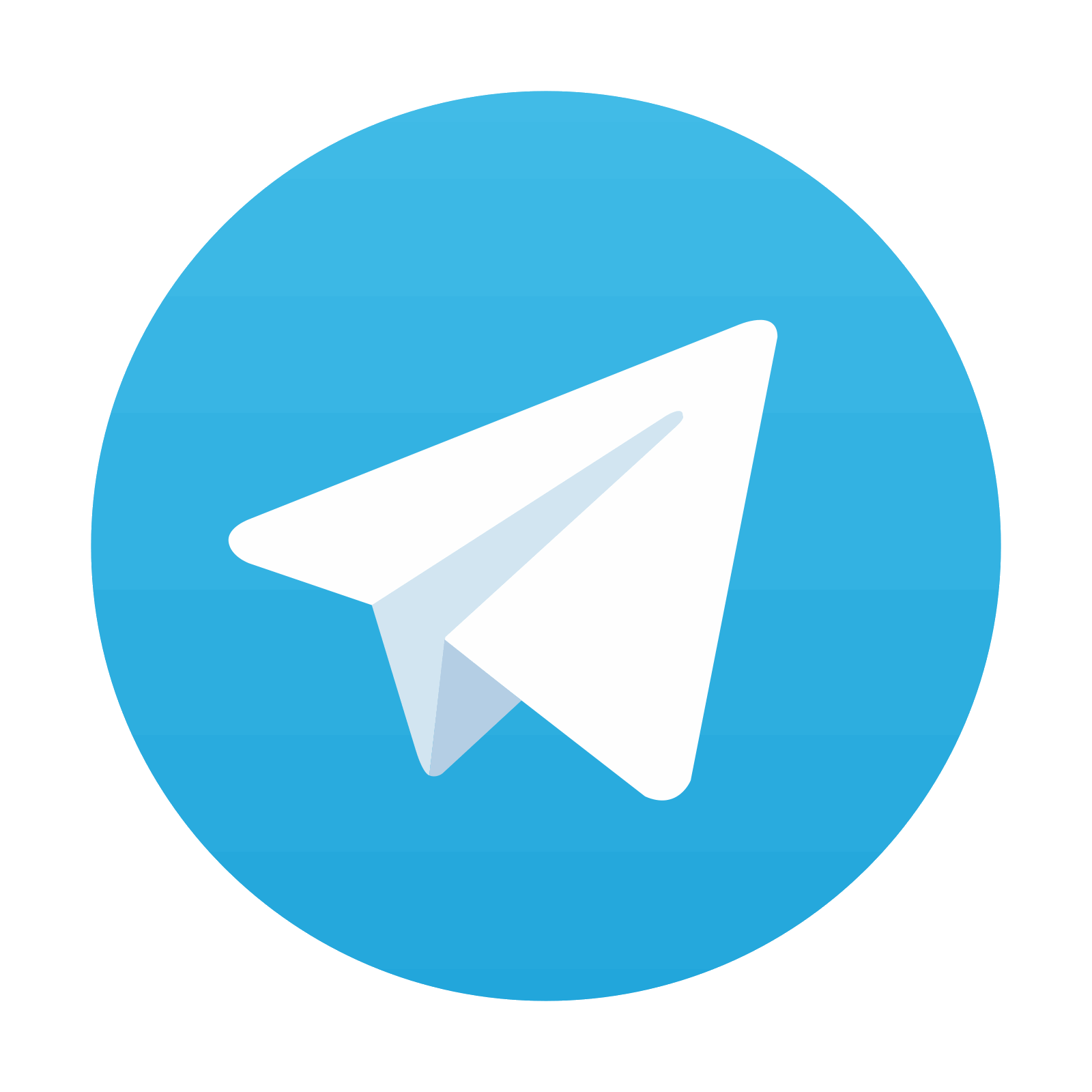
Stay updated, free articles. Join our Telegram channel
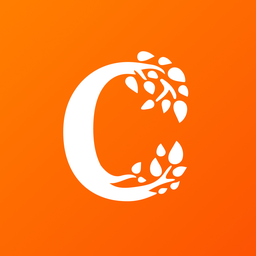
Full access? Get Clinical Tree
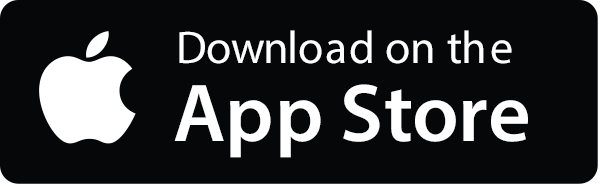
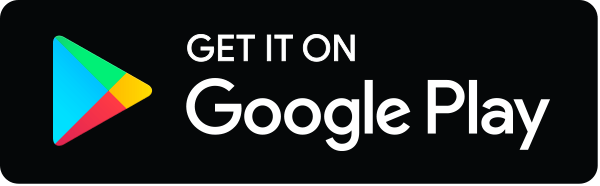