Abstract
Motor neurons (MNs) are a diverse group of cells without which life would not be possible. MNs are responsible for integrating signals from the brain and the sensory systems to control all voluntary and involuntary movement, along with parts of the autonomic nervous system. Here, we focus on the specification and maturation of spinal MNs as they are a key target of disease and injury, as well as regenerative efforts. MNs arise from progenitors in the ventral neural tube and migrate into columns and pools. Notably, these progenitors later generate oligodendrocyte precursor cells. MN axons then travel outside of the central nervous system to reach their targets under guidance cues from the extracellular matrix. These cells are targets of devastating diseases including amyotrophic lateral sclerosis, spinal muscular atrophy, multiple sclerosis, as well as spinal cord injuries. By decoding the major events and players in development, we can better recapitulate them in vitro for cell replacement therapy, or harness the underlying principles for regeneration in the adult.
Keywords
Neuronal development, spinal cord patterning, motor neuron development, embryonic stem cells, induced pluripotent stem cells, oligodendrocyte progenitor cells, pMN progenitor, hb9, olig2
Outline
Introduction 2
Specification of Neuroectoderm 3
Spinal Cord Patterning 3
The Motor Neuron Progenitor Domain and Initial Neurogenesis 5
Molecular Programs in Newborn Motor Neurons 5
Migration 6
Motor Neuron Subtypes and Targets 6
Somatic Motor Neurons 7
Visceral Motor Neurons 8
Axon Targeting 9
Extracellular Matrix and the Nervous System 10
Collagen 10
Laminin 10
Fibronectin 11
Proteoglycans and Glycosaminoglycans 11
Chondroitin Sulfate Proteoglycans 12
Heparan Sulfate Proteoglycans 12
Hyaluronic Acid 12
Motor Neuron Cell Death 13
The Glial Switch 13
Generating Motor Neurons From Pluripotent Stem Cells 14
Generating Oligodendrocyte Precursor Cells From Pluripotent Stem Cells 17
Conclusion 17
References
Introduction
Motor neurons (MN) are a diverse group of cells without which complex life would not be possible. MNs are responsible for integrating signals from the brain and the sensory systems to control voluntary and involuntary movements. Though MNs can be split into cranial and spinal subsets, this chapter will focus on spinal MNs, as they are a key target of disease and injury. As such, MNs are the focus of regenerative efforts to alleviate these public health burdens. During late gastrulation and neurulation, the developing spinal cord, termed the neural tube, is patterned into distinct progenitor domains. MNs are specified from progenitors in the ventral neural tube. Once specified, newly born MNs are further specified into columns, pools, and subtypes, forming a unique topography. From these columns and pools, axons reach out to their targets under varying guidance cues. All MNs are cholinergic cells which integrate with the motor control circuit, the sensory system, and their outlying targets to control movement. MNs are unique in that their targets lie outside the central nervous system (CNS), meaning that they require novel methods for seeking out and synapsing on them. Here, we present an overview of MN differentiation and development. We will focus mainly on signaling events, transcription factor markers, and the extracellular matrix (ECM) as they pertain to MN development. These cells are targets of permanent and often deadly diseases including amyotrophic lateral sclerosis, spinal muscular atrophy, multiple sclerosis, and injuries such as spinal cord injury. Only by understanding how these cells progress through development can we understand how to treat these maladies which currently have little hope of a cure. Further, by decoding the major events and players in development, we can better recapitulate them in vitro for cell replacement therapy, or harness the underlying principles for regeneration in the adult. Given the growing importance of the MN–glia interaction in a number of neurodegenerative diseases, we will also discuss the initial specification of oligodendrocyte precursor cells (OPCs) in detail, as they share a common progenitor with MNs.
Specification of Neuroectoderm
Vertebrate embryos specify the ectoderm in late gastrulation. This germ layer will become the epidermis and the nervous system. The anterior neural ectoderm is distinguished from the epidermis by its inability to bind bone morphogenic proteins (BMPs) due to the inhibitors secreted from the Spemann–Mangold organizer region of the gastrula. These inhibitors—noggin, chordin, and follistatin—bind and neutralize the effects of BMPs, creating a permissive transcriptional environment for neural progression. Posteriorly, the neural plate is specified by fibroblast growth factors (FGFs) and Wingless-related integration site (Wnt) proteins that also suppress BMP activity. Additionally, retinoid signaling from the paraxial mesoderm specifies the cells of the future spinal cord. This newly specified neural plate then thickens as cells proliferate and invaginates through the convergent extension, forming the neural groove. The neural groove forms hinge points which will ultimately close to form the neural tube – the precursor for the entire CNS. For an in-depth review, see Massarwa et al.
Spinal Cord Patterning
The spinal cord is a two-way information conduit that connects the brain with the sensory and motor systems. To do this, it must generate a highly diverse set of neurons during development. The neural tube provides a three-dimensional template which is patterned by gradients of morphogens to generate this diversity. The early neural tube is composed of multipotent neural stem cells expressing Sex determining region Y box 1 (Sox1). The dorsal neural tube will generate cells linking the CNS to the sensory peripheral nervous system (PNS). The ventral neural tube will ultimately give rise to the motor control circuit responsible for controlling MNs. Bone morphogenetic proteins specify the dorsal portion of the neural tube, including neuronal subtypes involved in integration of the peripheral sensory nervous system. Ventrally, an initial wave of Sonic hedgehog (Shh) from the notochord patterns the cells into distinct progenitor domains. These domains arise due to cross-repressive actions of two types of transcription factors downstream of Shh signaling: Type I transcription factors are repressed at threshold Shh concentrations, while Type II are expressed below threshold Shh concentrations ( Fig. 1.1A ). The type I transcription factor paired box protein 6 (Pax6) represses the activity of type II homeobox protein Nkx2.1. Similarly, type II homeobox Nkx6.1 cross-represses developing brain homeobox 2 (Dbx2). The most ventral progenitor domain is the floor plate, which is induced to secrete Shh in a second wave of patterning, followed by the progenitor domains p3, pMN, p2, p1, and p0 ( Fig. 1.1B ). The combinatory actions of these two classes of proteins yield the five spatially distinct ventral progenitor domains.

The Motor Neuron Progenitor Domain and Initial Neurogenesis
The MN progenitor (pMN) domain is responsible for generating MNs. In mice and chick models, this domain is identified by the expression of the homeobox transcription factors Nkx6.1 and Pax6 and the basic helix–loop–helix (bHLH) oligodendrocyte transcription factor 2 (Olig2). Olig2 expression is obligate for MN specification, as Olig2 null mice fail to generate MNs. Initially, Olig2 plays a key role in progenitor proliferation; however, it also drives the expression of neurogenin 2 (Ngn2), a key neural determinant. The first murine MNs are born around E9.5. The homeobox transcription factor Nkx2.2, important for the glial switch and a marker for p0 cells, shows variable expression in humans compared to mouse and chick models: the human pMN domain appears to include both Olig2+/Nkx2.2− as well as Olig2+/Nkx2.2+ cells. This could potentially add to the diversity of human MNs.
Molecular Programs in Newborn Motor Neurons
As mentioned above, Olig2 drives Ngn2 expression. However, Ngn2 is ultimately responsible for cell cycle exit and neurogenesis, in direct contrast to the role of Olig2. Once Ngn2 protein levels surpass those of Olig2, cell cycle exit occurs and cells commit to the neuronal lineage. Olig2 binds and sequesters the MN transcription factor homeobox gene 9 (Hb9, also called MNX1), which is necessary for MN development. LIM homeobox gene Isl1 and LIM homeobox 3 (Lhx3) form a complex with the nuclear LIM interactor which suppresses interneuron fate and specifies MN. Along with Ngn2, this complex stimulates Hb9, which self-stimulates its own expression, while forming a positive feedback loop with Isl1. Isl1 and 2 work in concert to further specify MN cell fate. Lhx3 and Isl1 expressions are necessary for MN generation and the expression of cholinergic genes common to all MNs. However, little is known about potential negative feedback mechanisms in this differentiation process that would limit MN number and organ size. We will discuss this further in the Glial Switch section.
In summary, Shh secreted from notochord drives the expression of Pax6 and Nxk6.1, which in turn drive Olig2 expression. Olig2 expression delineates a mitotic pMN progenitor. Olig2 induces the expression of Ngn2, which is responsible for cell cycle exit, of Lhx3/Isl1 transcription factors, as well as MN-specific Hb9 in newborn, postmitotic MNs ( Fig. 1.2 ).

Migration
The topography of MNs is largely correlated with their function. MNs cluster in columns with similar transcription factor expression and like targets. Within muscle-innervating columns, there are MN pools which innervate specific muscle groups. In order to specify this topography, MNs must migrate away from the ventricular progenitor cells to their final destination in the ventral horn of the spinal cord. Newly born neurons detach from the epithelium and migrate radially to the medial and lateral areas of the neural tube. Critical to this migration is cadherin expression driven by beta and gamma catenin signaling in a Wnt-independent manner. In knockout models of either cadherin, MNs fail to properly align to their proper column, although their ultimate muscular targets are not disrupted. This implies that the stereotypic and highly organized topology of MNs is not a modulator of identity or function. The role of this highly specific organization has yet to be elucidated. Transcriptionally, the forkhead box P (Foxp) genes regulate cadherin expression for migration to occur. Specifically, the Foxp2/4 genes allow for the detachment of MN from the neuroepithelium by downregulating cadherin 2 and allow them to migrate toward their final location by further modulation. Although cell bodies migrate within the spinal cord, all MN soma are exclusively contained within the spinal cord. Recently, Isl1/2 has been shown to play an integral part in preventing the cell body from exiting the spinal cord. In knockout animals, MN soma successfully exited the spinal cord into the periphery. One potential mechanism is through the semaphorin–neuropilin repulsive signaling pathway common to axon guidance, as neuropilin was found to be regulated by Isl1/2. For an in-depth review of migration and topography, see Kania (2005).
Motor Neuron Subtypes and Targets
MNs that innervate similar regions of the body group together in columns with identical molecular properties. The rostrocaudal axis is specified externally by retinoic acid (RA) at the cervical and brachial regions and by growth differentiation factor (GDF11) and FGF8 at the thoracic and lumbar regions. Like much of the developing embryo, this rostrocaudal positional identity is specified internally by Hox gene activation in response to these external cues to delineate the types of MNs in a given region. Hox expression is summarized in Fig. 1.3 . Hoxc9 plays a critical role in the organization of the spinal cord by repressing limb-specific Hox genes, thus specifying the thoracic column. Within each column, MNs organize into pools which innervate distinct muscles. Hox genes work in concert with the Hox accessory factor Foxp1 to specify many of the columns and establish motor pools within columns. Notably, in the absence of Foxp1 in knockout animals, there is a lack of defined rostrocaudal motor columns, further strengthening the case for its role in the positional identity of motor columns.

Somatic Motor Neurons
MNs can be separated into somatic and visceral motor columns based on their targets. Somatic MNs synapse directly onto muscles, including the body wall, limbs, and diaphragm. These include the phrenic motor column, medial motor column (MMC), hypaxial motor column, spinal accessory column, and lateral motor column (LMC). The variation in transcription factors expressed in these different columns most likely correlate to function. However, much work is needed to fully understand what the initial cues are that trigger these regulators and how they can account for such a diverse set of cells.
The phrenic motor column, located cervically, controls the diaphragm and respiratory actions. This column is specified by Isl1/2, Hb9, and homeobox gene Pou3F1 under the control of Hox5.
The spinal accessory motor column is located cervically and controls the muscles of the jaw and neck. These neurons are specified under the homeobox transcription factors Nkx9.2 and Nkx2.2 and require expression of paired like homeobox 2b (Phox2b).
MMC neurons innervate the muscles of the back and are unique in that they are present throughout the spinal cord, not being restricted to one region or under the influence of Hox genes. These cells in this column all express Hb9, Isl1/2 and are the only neurons to retain Lhx3 expression after specification. Lhx3 is thought to play an important role in overcoming Hox regionalization. These neurons also do not express Foxp1, which likely aids in escaping Hox control.
Body wall and abdominal muscles are innervated by the hypaxial motor column, located only in the thoracic region. These cells express Hb9, Isl1, Ets variant 1 (Etv1), and low levels of Isl2, while being negative for Foxp1. Like the MMC, the lack of Foxp1 expression likely aids in their ability to escape Hox regionalization in order to span a large part of the spinal cord.
Limb-innervating neurons are found in either the brachial or lumbar region in the LMC. This can be further subdivided into the medial (LMCm) and lateral (LMCl) regions that innervate ventral and dorsal muscles, respectively, during development. Further specification includes the type of muscle innervated. These cells express Isl2, Foxp1, and the aldehyde dehydrogenase family gene Aldh1A2, a protein involved in RA synthesis, while downregulating Lhx3. Recently, the Onecut transcription factor family has been implicated in LMCm and LMCl fate decisons. Conditional dicer knockouts result in a severe reduction in LMC MNs through apoptosis, implicating miRNA as a key determinant in their survival.
There are three types of MNs located within a pool synapsing on a specific muscle group: alpha, beta, and gamma. Alpha MNs innervate extrafusal muscles and cause muscle contraction, including reflex responses, synapsing on fast-twitch fatigable, slow-twitch fatigue-resistant, and fast-twitch fatigue-resistant fibers. Beta MNs innervate both extra- and intrafusal muscle fibers, though their identity and role are poorly understood. Gamma MNs synapse on intrafusal fibers to modulate muscle sensitivity. Gamma MNs are a distinct subset and can be identified by the secretion of Wnt7a.
Visceral Motor Neurons
As opposed to somatic MNs, visceral MNs regulate the autonomic nervous system and are found in the thoracic level of the spinal cord in the preganglionic column. These are unique in that they do not synapse on muscles, but rather the ganglia of the PNS. Through this, they are able to regulate smooth muscle, cardiac muscle, and glandular activity. These cells express mothers against decapentaplegic homolog 1 (Smad1), nitric oxide synthase 1, Smad interacting protein 1 (Sip1), and low levels of Foxp1. The presence of Sip1 and Smad1 imply an important role for BMPs, possibly by distinguishing them from the somatic MNs during specification. Isl2 is downregulated to specify this cell type. Recently, the Onecut family of factors which drive Isl1 were revealed to play an important role in visceral/somatic fate decisions. In the more caudal regions, preganglionic motor column neurons regulate the parasympathetic system by projecting to ganglia near their target organs, while in the thoracic regions, they synapse on the ganglia in proximity to the spine to regulate the sympathetic systems of the body.
Axon Targeting
MN axons must exit the CNS in order to project onto their targets. A small subset of MNs, specifically the spinal accessory column, exit dorsally through the lateral exit point. These neurons express the netrin receptor, and are repelled from the midline of the spinal cord which expresses netrin-1. However, most MN axons exit ventrally, driven by the chemokine C-X-C motif receptor 4 (Cxcr4) expressed on the neurons and its ligand chemokine C-X-C ligand 12 (Cxcl12) located in the ventral paraxial mesoderm. Once the axons have exited, they are directed toward the body wall, the limbs, the muscles of the back, or the autonomic ganglia, which will ultimately determine which motor column they will become. MMC MNs express ephrinA 3 and 4 receptors (EphA3/4) which repel them from the ephrin-A1 (Efna1)-secreting dorsal root ganglia to continue to the back muscles. These neurons express FGF receptor 1, which is also thought to direct them. LMCs, the limb-innervating MNs, use a semaphorin–neuropilin complex in timing the invasion of the limb. Once they have reached the limb bud, LMCl neurons express EphA4 receptor. Ephrins in the ventral limb repel these neurons and they extend to the dorsal limb. LMCm neurons express EphB1 and are similarly repelled from the dorsal limb which expresses ephrinB. Though many of the mechanisms are poorly understood, understanding how axons migrate to their targets will be important for cell replacement therapies.
Once axons reach their target muscle, extrinsic factors play a critical role in finalizing the connections. Muscle-derived glial cell line-derived neurotrophic factor (GDNF), specifically, drives Ets variant 4 (Etv4) which induces MNs to arborize and innervate muscle fibers. The Onecut gene family involved in MN specification has additionally been identified as a transcriptional regulator of neuromuscular junction formation. For a review on axon guidance, see Stifani.
Extracellular Matrix and the Nervous System
The ECM of the nervous system plays an important role in facilitating neuronal migration and axonal pathfinding during CNS and PNS development. The nervous system ECM is compositionally distinct, consisting of markedly lesser quantities of fibrous proteins such as collagens, and fibronectins, and displaying a higher concentration of glycoproteins and proteoglycans when compared to the ECM of other systemic tissues. The contribution of CNS and PNS ECM components to neuronal function and homeostasis, emphasizing MNs, are presented in this section.
Collagen
In contrast with other tissue-specific ECMs, collagen type IV is the only collagen found in the CNS ECM. A majority of the collagen IV in the CNS ECM self-polymerizes along with fibronectin and laminin to form the basement membrane, which serves as a protective barrier between the vascular endothelial cells and the parenchyma of the brain. Collagen IV is also associated with the neural interstitial matrix, where it is found in small amounts along with small amounts of other such adhesive glycoproteins such as fibronectin and laminin, and sulfated glycoproteins such as entactin. These minor components are interlinked within a major network of chondroitin sulfate proteoglycans (CSPGs), hyaluronic acid (HA), and tenascin, which make up the bulk of the neural interstitial matrix.
Collagens in the PNS are broadly classified into the fibrillary type I, III, and V collagens and the basement membrane associated type IV collagen. The fibrillary collagens play an important role in supporting myelinating Schwann cell function. Long-term (14 to 28 days) in vitro cultures of MNs in 3D type I and III collagen scaffolds produced elongated neurites of ~850 µm in length with thick myelin sheaths, indicating that trophic support provided by fibrillary collagens is essential for MN growth. On the contrary, dysregulated fibrillary collagen associated with scar tissue formed after PNS injury is considered to be a major barrier to nerve regeneration.
Laminin
Laminins are large (~800 kDa) ECM-associated glycoproteins thought to be essential for neuronal migration and axonal pathfinding during development. They are heterotrimeric molecules consisting of α, β, and γ chains. These chains present themselves in a variety of different combinations to result in as many as 18 distinct laminin isoforms, a majority of which are present in the nervous system ECM. S-laminin is a homolog of the B1 subunit of laminin. It is known to facilitate MN adhesion, and plays an important role in the formation of neuromuscular junctions. In the developing CNS, S-laminin and laminin are both found to be present in the subplate in the cerebral cortex. This situation changes dramatically in the adult CNS, which is marked by the disappearance of S-laminin, and the restricted expression of laminin to the basement membrane, where it is found in close association with collagen and heparan sulfate proteoglycans (HSPGs).
Laminins are important constituents of the PNS basement membrane found surrounding Schwann cells. As integral adhesive and growth-promoting constituents of the basement membrane, they are believed to organize together the meshwork of structural proteins to form the substratum upon which neuronal migration and axonal pathfinding can take place. The diversity of laminin isoforms can be advantageous in regulating the selective attachment of MNs, which is reportedly mediated by the S-laminin-specific LRE (leucine–arginine–glutamic acid) amino acid sequence.
Fibronectin
Fibronectins are ECM proteins known to play specific roles in neuronal migration during development and after injury. Like laminin, they are large glycoproteins consisting of functional domains that facilitate cellular and ECM interactions. In the CNS they are associated with the basement membrane where they are known to interact with collagen, HSPGs, and tenascin. Fibronectin in the PNS is specifically upregulated after injuries. This is also accompanied by the upregulation of α 5 β 1 integrin on the cell membranes of regenerating MNs and Schwann cells, which is necessary for blood vessel development, pointing to the role of fibronectin in facilitating PNS repair.
Proteoglycans and Glycosaminoglycans
Proteoglycans such as CSPGs and HSPGs, and their associated sulfated glycosaminoglycans (GAGs), as well as unsulfated GAGs such as HA constitute the majority of the CNS ECM. High-molecular-weight HA forms the diffuse meshwork to which CSPGs, HSPGs, tenascins and other fibribrous ECMs proteins are linked. This network is more condensed around presynaptic terminals, nodes of Ranvier, and around the perineuronal nets that surround inhibitory interneurons.
Chondroitin Sulfate Proteoglycans
Sulfated CSPGs belonging to the lecitican family (aggrecan, versican, neurocan, and brevican) are the most abundant CSPGs associated with the CNS ECM and play a major role in regulating neuronal plasticity. They are large proteins consisting of multiple domains capable of specifically binding HA, tenascin, and other ECM components. The associated CS-GAG sidechains can be sulfated at various positions, resulting in a complex configuration of sulfated GAGs that are capable of mediating a variety of different functions, some of which include receptor and growth factor binding. The oversulfated CS-GAG CS-4,6 sulfate (CS-E) is a potent neurite repellant that is specifically upregulated after CNS injury. Nerve repulsive CSPGs are also believed to regulate axonal regeneration following peripheral neuron crush injury, where they are found to bind and inhibit the growth-promoting activity of laminin.
Heparan Sulfate Proteoglycans
In contrast to the predominantly nerve-repulsive role played by CSPGs, large basement-membrane-associated HSPGs such as perlecan are known to complex with laminin to induce neurite outgrowth. However, in the context of neurodegenerative diseases such as Alzheimer’s disease, HSPGs are thought to exacerbate the formation of amyloid-β and prevent its proteolytic degradation. In the PNS, HSPGs such as glypican-1 are upregulated in the dorsal root ganglia after sciatic nerve transection, where it is believed to promote target MN reinnervation.
Hyaluronic Acid
HA is an unsulfated GAG that interacts with CD44 and other cell surface receptors in the brain ECM. HA is abundantly found in the neural interstitial matrix where it is complexed with CSPGs. The upregulation of HA in response to CNS injuries is believed to inhibit remyelination of neurons. However, the role of HA in directly influencing these outcomes remains to be elucidated owing to the fact that it is often bound to CSPGs. Recent studies have indeed demonstrated that the enzymatic degradation of HA by the hyaluronidase PH20, but not other hyaluronidases, results in digestion products that can further impede remyelination. In the PNS, HA is found to be densely deposited at the nodes of Ranvier in peripheral neuron axons. Previous studies evaluating the regenerative potential and function of transected sciatic nerves treated with HA report significant improvements in nerve conduction velocity and muscle mass, and a significant reduction in scarring when compared to saline-treated animals. These seemingly contradictory functions point to the diverse roles played by HA in the CNS and PNS, and also highlight the importance of the role of enzymatic degradation of these GAGs in regulating these end outcomes.
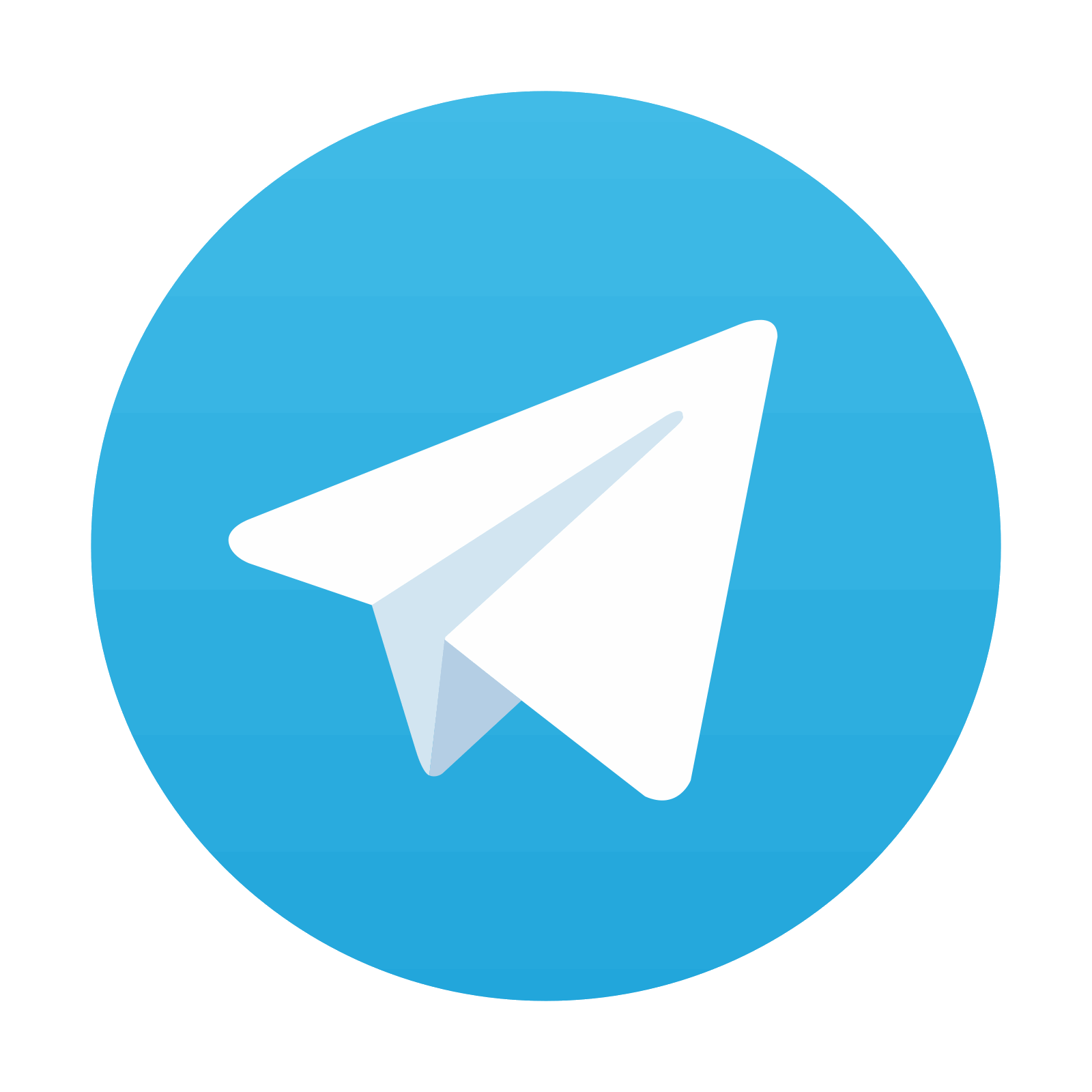
Stay updated, free articles. Join our Telegram channel
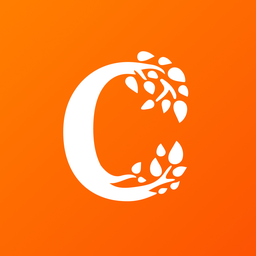
Full access? Get Clinical Tree
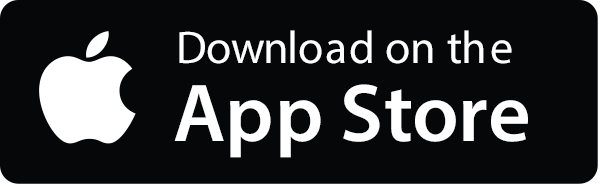
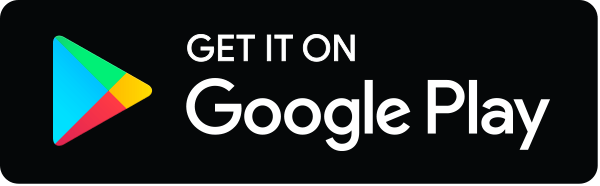