This article provides historical background and current research involving the use of bevacizumab for the treatment of recurrent glioblastoma. Although bevacizumab, approved by the Food and Drug Administration, prolongs glioblastoma progression free survivial, decreases tumor vascularization, and reduces permeability of vessels, it does not seem to prolong overall survival. Despite slowed primary tumor progression, bevacizumab treatment may facilitate transformation to a more invasive phenotype. Adaptive responses, which make glioblastoma particularly resistant to various treatment modalities have been described. Conferred benefits, adverse effects, mechanisms of resistance, and potential areas for future research are discussed.
- •
The overall benefit of bevacizumab remains controversial.
- •
Although bevacizumab has been shown to extend progression-free survival, it has not been shown to improve overall survival and may facilitate glioma transformation to a more invasive phenotype.
- •
The mechanism of bevacizumab is still not sufficiently understood and future studies may need to use novel methods of evaluating and visualizing tumor progression to determine the effectiveness of bevacizumab.
Introduction
Glioblastoma multiforme (GBM) is one of the most common and aggressive primary brain tumors. Despite surgical resection, radiotherapy, and chemotherapy, prognosis for GBM remains poor. The median progression-free survival (PFS) and overall survival (OS) for patients with GBM who undergo surgical resection followed by radiation therapy and temozolomide chemotherapy are 6.9 and 14.7 months, respectively.
GBM is a highly invasive and one of the most angiogenic and vascularized cancer. Altered pathways in GBM include the loss of function of tumor suppressor genes and the activation of oncogenes. GBM is believed to be characterized by tumor progression through the induction of angiogenesis to form new blood vessels via endothelial cell migration and proliferation. GBM is capable of exhibiting endothelial proliferation and rapid formation of tortuous vessels to supply its increasing metabolic needs; as a result, its poor-quality blood vessels are highly permeable and disorganized. Angiogenesis may play a pivotal role beginning in the earliest phase of tumor development and perhaps represents a critical event in the progression of malignant gliomas. Hence, angiogenesis has been targeted in the treatment of recurrent GBM.
Recurrent Glioblastoma
Nearly all GBM recur after initial therapy, and only 20% to 25% of patients survive beyond 1 year after the diagnosis of recurrent disease. Median survival for patients with recurrent GBM ranges from 3 to 9 months. Several potential treatments are being evaluated in ongoing clinical trials for recurrent GBM. bevacizumab (Avastin), a humanized anti-vascular endothelial growth factor (VEGF) antibody, has shown promising results in phase II clinical trials for recurrent GBM; as a result, in May 2009, the Food and Drug Administration (FDA) approved the use of bevacizumab for the treatment of patients with recurrent GBM, which made bevacizumab the third FDA-approved chemotherapy for GBM along with implantable Gliadel wafers and temozolomide.
Bevacizumab
Since its inception, bevacizumab has led a successful yet controversial path. Initially indicated for metastatic colorectal cancer in 2004, bevacizumab was shown in a randomized, double-blind, stage III clinical trial to significantly extend both PFS and duration of survival. Median PFS in a colorectal cancer control group receiving IFL (irinotecan, fluorouracil, and leucovorin) treatment was 6.2 months, compared with 10.6 months in a group receiving IFL with bevacizumab. Median duration of survival increased from 15.6 months in the IFL control group to 20.3 months in the group receiving IFL and bevacizumab. Because of its versatility and success in treating colorectal cancer, bevacizumab was considered as the first anti-angiogenic therapy approved in the United States. Bevacizumab later gained approval for use in several other solid tumors, including lung cancer, breast cancer, renal cell cancer, and in 2009, was granted accelerated approval for GBM.
However, in 2010, the FDA rescinded its approval of bevacizumab for metastatic breast cancer based on the lack of evidence for improved OS in phase III clinical trials when compared with standard anti-mitotic chemotherapies, such as docetaxel, 5-fluorouracil, epirubicin, and cyclophosphamide. An additional point of concern was the severity of the side effects, including wound dehiscence, gastrointestinal perforation, hemorrhage, and high-grade thrombosis. However, the other indications remain and bevacizumab continues to be evaluated as a promising treatment in clinical trial for patients with recurrent GBM. Based on these results, only in GBM is bevacizumab approved as a single agent.
Although bevacizumab prolongs GBM PFS, decreases tumor vascularization, and reduces permeability of vessels, it does not prolong the OS. Bevacizumab alone gives a median PFS of 4 months and 6-month PFS (PFS6) of 29%. A meta-analysis of 548 patients showed that bevacizumab given in combination with other drugs gives a higher PFS6 rate of 45%, a 6-month OS rate of 76%, and an OS of 9.3 months. Another study comparing bevacizumab with combination bevacizumab and irinotecan therapy showed that PFS6 is higher when bevacizumab is taken with irinotecan (PFS6 of 50.2% for combination vs 35.1% for bevacizumab alone). Despite the clear benefit of irinotecan plus bevacizumab on PFS, OS for bevacizumab plus irinotecan is 8.9 months, although bevacizumab alone only marginally extends OS to 9.7 months. This lack of a clear benefit in OS for one therapy over another adds to the uncertainty of the benefit of anti-angiogenic therapies.
Subsequently, the FDA’s approval of bevacizumab for the treatment of recurrent GBM was accelerated with PFS being relied on as a metric for drug efficacy rather than OS. However, the validity of PFS as an accurate measurement of treatment outcome has been questioned. Although statistical analysis shows that PFS and OS are strongly associated, it has been argued that PFS is not a replacement for OS and cannot be predictive of tumor growth after treatment.
Furthermore, several studies suggest that 1 adverse consequence of this pharmacologic treatment includes hijacking of healthy nontumorous vasculature. Vessels from normal tissue are recruited via tumor infiltration to supply the lesion in a process known as co-option. In short, the mechanism of bevacizumab is still not sufficiently understood and must be further elucidated to evaluate its far-reaching effects.
Molecular mechanism of bevacizumab
In theory, the mechanism of bevacizumab is exquisitely simple. As an antibody for angiogenic factors, bevacizumab cuts off a tumor’s life supply by inhibiting the factors required to promote and sustain vessel growth. Angiogenesis has been extensively studied for decades. Tumors should theoretically stop growing if vascularization is absent or insufficient. However, this mechanism has proven to be more difficult in clinical practice than expected, and nonangiogenic pathways that may be affected by bevacizumab are also under scrutiny.
Angiogenesis, or sprouting of new vessels from parent vessels, first involves vascular endothelial breakdown and then proliferation of existing vasculature. Extensive investigation has revealed several VEGF-dependent and VEGF-independent pathways that regulate angiogenesis. VEGF is recognized as one of the most potent stimuli for angiogenesis, making it a key pathway of deregulation in GBM development and proliferation.
VEGF
VEGF, also known as VPF (vascular permeability factor), comprises a family of 5 proteins (VEGF-A, VEGF-B, VEGF-C, VEGF-D, and placental-derived growth factor) that regulate vasculogenesis during growth, lymphatic development, wound healing, menstruation, and pregnancy. Hypoxia commonly induces VEGF release, and in particular, VEGF-A is released by GBM as a mitogen that accumulates in nearby blood vessels. After release from hypoxic malignant cells, VEGF binds to both pericytes and vascular endothelial cells.
Pericytes typically wrap around the outside surface of vascular endothelial cells to maintain stability of the blood-brain barrier. In mice without pericytes, extravasation occurs as intravenously administered tracers are found to seep out of vessels and into brain tissue. In fact, the proper functioning of the blood-brain barrier depends on the extent of pericyte coverage of vessels. In the presence of VEGF, pericytes detach from the vessel wall, resulting in a weakened vessel basement membrane and contributing to the compromised blood-brain barrier that is often observed in proximity to gliomas.
In addition to binding to pericytes, VEGF-A binds to the surface of vascular endothelial cells through either vascular endothelial growth factor receptor (VEGFR) -1 (also known as Flt-1) or VEGFR-2 (also known as KDR or Flk-1). The binding of VEGF-A to VEGFR-2 produces tyrosine kinase activity that is 10 times more potent than the activity of VEGF-B to VEGFR-1 binding. Not coincidentally, bevacizumab targets VEGF-A and neutralizes its many downstream effects. For endothelial cell proliferation, the VEGFR-2 signal transduces through phospholipase C to C-Raf-MAP kinase. For endothelial cell survival and migration, the VEGFR-2 signal transduces through a tyrosine kinase PI3 K-Akt cascade to activate focal adhesion kinase. One of the first cellular changes observed with VEGF binding is a 4-fold increase in intracellular calcium, which may be used as a marker for VEGF pathway activation.
One of several VEGF downstream pathways leads to the phosphorylation of occludin and zonula occludens-1, key proteins of tight junction function and organization. Phosphorylation ultimately causes the gap junctions between endothelial cells to loosen, resulting in fenestrations, vessel dilation, and additional increase in vascular permeability. Permeability allows proteins to extravasate and extracellular matrix to be laid down as the foundation for new vessels. Endothelial cells recruit to the new extracellular matrix, and the cell at the tip of the growing endothelial cell group (known as the tip cell) is responsible for sensing environmental cues to direct vessel growth. HIF-1α (hypoxia-inducible factor) sensitizes these endothelial cells to angiogenic factors. VEGF-A induces vascular hyper-permeability and sprouting of new vessels from preexisting vasculature. Therefore, the hyperplastic vascularization that is characteristic of GBM may be because of the high levels of VEGF.
Dvorak and colleagues argue that movement of fluid between endothelial cell tight junctions and compromised endothelial cells is not sufficient to account for all of the extravasation that occurs in the presence of VEGF. Instead, they report that an intracellular system, the vesicular-vacuolar organelle (VVO), is responsible for VEGF-induced microvascular permeability. More organized than caveolae, VVOs are a system of vesicles or vacuoles that transport fluid and molecules across endothelial cells. Moreover, VVO function upregulates in the presence of VEGF ( Fig. 1 ).
Upregulation of VEGF in Glioblastoma
In GBM, VEGF-A is upregulated due to hypoxia and necrosis induced by rapid tumor growth. The gene sequence for VEGF shares common elements with erythropoietin, which is also transcribed in an oxygen-level–dependent manner. The stress of unmet perfusion demands (resulting in decreased oxygen, reduced nutrients, and insufficient blood flow) induces the release of VEGF. Cells must always be near nutritional support, otherwise necrosis occurs. Hypoxia stabilizes HIF-1α and HIF-2α, which promote metabolic changes to sustain continued tumor growth. Specifically, HIF-1α and -2α have the following effects: (1) block the proteolysis of VEGF by ubiquitin, therefore allowing VEGF to accumulate ; (2) upregulate GLUT-1 receptors to increase uptake of glucose from blood ; (3) upregulate carbonic anhydrase IX (CAIX) to stabilize pH in hypoxic conditions, making the environment tolerable for continued proliferation ; and (4) sensitize endothelial cells to angiogenic signals. One group suggests that different degrees of hypoxia induce different sets of rescue proteins: mild hypoxia induces the production of HIF-1α and VEGF, whereas severe hypoxia induces the production of CAIX. Ultimately, this results in growth of additional vessels to supply the malignancy with the nutrients needed to support continued growth. All of these adaptive responses make GBM particularly resistant to various treatment modalities.
Molecular mechanism of bevacizumab
In theory, the mechanism of bevacizumab is exquisitely simple. As an antibody for angiogenic factors, bevacizumab cuts off a tumor’s life supply by inhibiting the factors required to promote and sustain vessel growth. Angiogenesis has been extensively studied for decades. Tumors should theoretically stop growing if vascularization is absent or insufficient. However, this mechanism has proven to be more difficult in clinical practice than expected, and nonangiogenic pathways that may be affected by bevacizumab are also under scrutiny.
Angiogenesis, or sprouting of new vessels from parent vessels, first involves vascular endothelial breakdown and then proliferation of existing vasculature. Extensive investigation has revealed several VEGF-dependent and VEGF-independent pathways that regulate angiogenesis. VEGF is recognized as one of the most potent stimuli for angiogenesis, making it a key pathway of deregulation in GBM development and proliferation.
VEGF
VEGF, also known as VPF (vascular permeability factor), comprises a family of 5 proteins (VEGF-A, VEGF-B, VEGF-C, VEGF-D, and placental-derived growth factor) that regulate vasculogenesis during growth, lymphatic development, wound healing, menstruation, and pregnancy. Hypoxia commonly induces VEGF release, and in particular, VEGF-A is released by GBM as a mitogen that accumulates in nearby blood vessels. After release from hypoxic malignant cells, VEGF binds to both pericytes and vascular endothelial cells.
Pericytes typically wrap around the outside surface of vascular endothelial cells to maintain stability of the blood-brain barrier. In mice without pericytes, extravasation occurs as intravenously administered tracers are found to seep out of vessels and into brain tissue. In fact, the proper functioning of the blood-brain barrier depends on the extent of pericyte coverage of vessels. In the presence of VEGF, pericytes detach from the vessel wall, resulting in a weakened vessel basement membrane and contributing to the compromised blood-brain barrier that is often observed in proximity to gliomas.
In addition to binding to pericytes, VEGF-A binds to the surface of vascular endothelial cells through either vascular endothelial growth factor receptor (VEGFR) -1 (also known as Flt-1) or VEGFR-2 (also known as KDR or Flk-1). The binding of VEGF-A to VEGFR-2 produces tyrosine kinase activity that is 10 times more potent than the activity of VEGF-B to VEGFR-1 binding. Not coincidentally, bevacizumab targets VEGF-A and neutralizes its many downstream effects. For endothelial cell proliferation, the VEGFR-2 signal transduces through phospholipase C to C-Raf-MAP kinase. For endothelial cell survival and migration, the VEGFR-2 signal transduces through a tyrosine kinase PI3 K-Akt cascade to activate focal adhesion kinase. One of the first cellular changes observed with VEGF binding is a 4-fold increase in intracellular calcium, which may be used as a marker for VEGF pathway activation.
One of several VEGF downstream pathways leads to the phosphorylation of occludin and zonula occludens-1, key proteins of tight junction function and organization. Phosphorylation ultimately causes the gap junctions between endothelial cells to loosen, resulting in fenestrations, vessel dilation, and additional increase in vascular permeability. Permeability allows proteins to extravasate and extracellular matrix to be laid down as the foundation for new vessels. Endothelial cells recruit to the new extracellular matrix, and the cell at the tip of the growing endothelial cell group (known as the tip cell) is responsible for sensing environmental cues to direct vessel growth. HIF-1α (hypoxia-inducible factor) sensitizes these endothelial cells to angiogenic factors. VEGF-A induces vascular hyper-permeability and sprouting of new vessels from preexisting vasculature. Therefore, the hyperplastic vascularization that is characteristic of GBM may be because of the high levels of VEGF.
Dvorak and colleagues argue that movement of fluid between endothelial cell tight junctions and compromised endothelial cells is not sufficient to account for all of the extravasation that occurs in the presence of VEGF. Instead, they report that an intracellular system, the vesicular-vacuolar organelle (VVO), is responsible for VEGF-induced microvascular permeability. More organized than caveolae, VVOs are a system of vesicles or vacuoles that transport fluid and molecules across endothelial cells. Moreover, VVO function upregulates in the presence of VEGF ( Fig. 1 ).
Upregulation of VEGF in Glioblastoma
In GBM, VEGF-A is upregulated due to hypoxia and necrosis induced by rapid tumor growth. The gene sequence for VEGF shares common elements with erythropoietin, which is also transcribed in an oxygen-level–dependent manner. The stress of unmet perfusion demands (resulting in decreased oxygen, reduced nutrients, and insufficient blood flow) induces the release of VEGF. Cells must always be near nutritional support, otherwise necrosis occurs. Hypoxia stabilizes HIF-1α and HIF-2α, which promote metabolic changes to sustain continued tumor growth. Specifically, HIF-1α and -2α have the following effects: (1) block the proteolysis of VEGF by ubiquitin, therefore allowing VEGF to accumulate ; (2) upregulate GLUT-1 receptors to increase uptake of glucose from blood ; (3) upregulate carbonic anhydrase IX (CAIX) to stabilize pH in hypoxic conditions, making the environment tolerable for continued proliferation ; and (4) sensitize endothelial cells to angiogenic signals. One group suggests that different degrees of hypoxia induce different sets of rescue proteins: mild hypoxia induces the production of HIF-1α and VEGF, whereas severe hypoxia induces the production of CAIX. Ultimately, this results in growth of additional vessels to supply the malignancy with the nutrients needed to support continued growth. All of these adaptive responses make GBM particularly resistant to various treatment modalities.
VEGF and bevacizumab
By neutralizing VEGF-A, bevacizumab induces tumor hypoxia and also blocks the mechanism by which new vessels are induced. Although circulating VEGF levels increase after the administration of VEGF antibodies, the compensatory upregulation is not enough to induce substantial angiogenesis. In rats bearing human GBM xenografts, it is observed that the tumor is subjected to hypoxia, resulting in reduced growth, decreased mitochondria, and diminished edema caused by the decreased permeability of vessels.
Tumors treated with bevacizumab do not exhibit the normal histologic characteristics of GBM. Bevacizumab successfully stops proliferation of vessel endothelium and pseudopalisading necrosis. Fewer mitochondria are present within cells, and microareas of cell death, as suggested by the presence of cell lysis, are seen in the core of the tumor. However, the edges of the tumor uniquely comprises loosely connected cells that may suggest increased invasiveness, a potentially harmful consequence of bevacizumab treatment.
Bevacizumab inhibits the VEGF-induced permeability of vessels. Treatment with bevacizumab shows a marked decrease in edema as early as 1 day after treatment according to a phase II trial, therefore replacing the need for corticosteroids given to block transendothelial fluid flow by regulating endothelial tight junctions. Reduction in edema occurs in 50% of patients, equating to a 59% reduction in corticosteroid doses. As a result, minimized corticosteroid administration allows for a decrease in complications, mortality, and morbidity associated with its chronic use. In a phase II study, bevacizumab significantly decreased abnormal fluid attenuated inversion recovery (FLAIR) signal by more than 5% in 77% of patients; 79% of patients had more than 5% volume reduction on T1-weighted magnetic resonance imaging (MRI) with contrast enhancement. Another study reported that 93.2% of patients showed radiographic response (including complete response, partial response, and minimal response) and 34.1% showed either complete or partial response. T2-weighted MRI taken after bevacizumab treatment shows substantial reduction in contrast enhancement. Similar results are seen in FLAIR signals with bevacizumab treatment. However, this apparent improvement according to MRI does not necessarily correspond to reduction in activity of the tumor and may simply be because of the reduction in the vascular permeability of gadolinium, which gives an exaggerated impression of tumor reduction. According to a study comparing changes before and after bevacizumab treatment in T2-weighted MRI and contrast-enhancing volume, there was no significant correlation between tumor volume and PFS or OS ( Table 1 ).
Tumor Region of Interest | PFS ( P -Value) | OS ( P -Value) |
---|---|---|
Pretreatment T2 volume | 0.2909 | 0.7226 |
Posttreatment T2 volume | 0.8385 | 0.6421 |
Change in T2 volume | 0.1624 | 0.5882 |
Pretreatment CE volume | 0.3142 | 0.7963 |
Posttreatment CE volume | 0.6914 | 0.5547 |
Change in CE volume | 0.0760 | 0.4392 |
However, there is a significant linear correlation between proliferation rate and PFS. In addition, infiltrative GBM after bevacizumab treatment is often nonenhancing, making radiographic evaluation difficult and misleading. Overall, pseudoresponse confounds interpretation of imaging after bevacizumab use and may skew the apparent effectiveness of bevacizumab on tumor size and disease progression. As the glioma response to bevacizumab is difficult to assess with traditional computed tomography and MRI, different imaging methods may be necessary to properly evaluate the benefits of this therapeutic approach.
As the concentration of VEGF correlates with vessel density, anti-VEGF therapy reduces concentrations of active VEGF and leads to a subsequent decrease in vessel density. Staining of endothelial cells after bevacizumab administration shows that large-sized vessels are reduced by 58%, whereas medium-sized vessels are reduced by 17%. However, there is no change in small-sized vessels, indicating that limits exist in vessel remodeling and destruction. This also suggests the role of other angiogenic pathways in maintaining blood flow to the tumor. In addition, bevacizumab works very rapidly; as visualized by CD34 immunohistochemistry, tumor vessels drop to less than 50% of control density by 1 day after administration, and less than 30% of control vessel density by day 7.
Because of the reduction in vessel density, bevacizumab induces a hypoxic state in tumors, resulting in the accumulation of lactate, alanine, choline, myo-inositol, creatine, taurine, mobile lipids, and HIF-1α. Although HIF-1α is involved with critical tumorigenic, survival, and angiogenic pathways in GBM, bevacizumab prevents vascularization associated with HIF-1α. As such, tumor upregulation of HIF-1α is not sufficient to induce substantial angiogenesis, yet the tumor finds other pathways for proliferation and invasion.
Although bevacizumab reduces vascularization of tumors, it also results in glioma transformation from an expansive to an invasive phenotype. One group was able to induce invasiveness from a xenograft glioma animal model of previously noninvasive tumor by treating with bevacizumab. The borders of bevacizumab-treated tumors are extremely invasive, spreading far beyond landmark vessels, in comparison with untreated controls. In addition, distant recurrences were common in patients treated with bevacizumab, even if they had reduced radiographic enhancement. It is possible that the physiologic stress of hypoxia and reduction in vascularization after anti-VEGF treatment leads to the highly invasive properties of the xenografts. Several other groups observed that when angiogenesis is inhibited, the tumor activates an alternate pathway that uses preexisting vessels. This bevacizumab-induced vessel co-option occurs with highly invasive tumors, resulting in a significant increase in satellite tumor area when compared with controls. However, primary tumor size does not change with anti-VEGF antibody, and growth rate slows. Therefore, despite slowed primary tumor progression, bevacizumab treatment may activate a separate highly invasive quality in satellite tumors that infiltrate healthy tissue to take over preexisting blood vessels. Co-option provides a VEGF-independent method of vascularization of tumors and therefore serves as a method of escape from anti-VEGF treatment.
Analysis of gene expression after bevacizumab therapy demonstrates an upregulation of the Wnt pathway indicating a potential mechanism for the differentiation and infiltrative nature of these gliomas after treatment. Similarly, bevacizumab induces more than half of the genes related to the PI3 K/Akt pathway to increase, resulting in pathway activation and reduction in apoptosis. In combination, these factors seem to promote tumor proliferation and invasion, despite anti-angiogenic treatment effects.
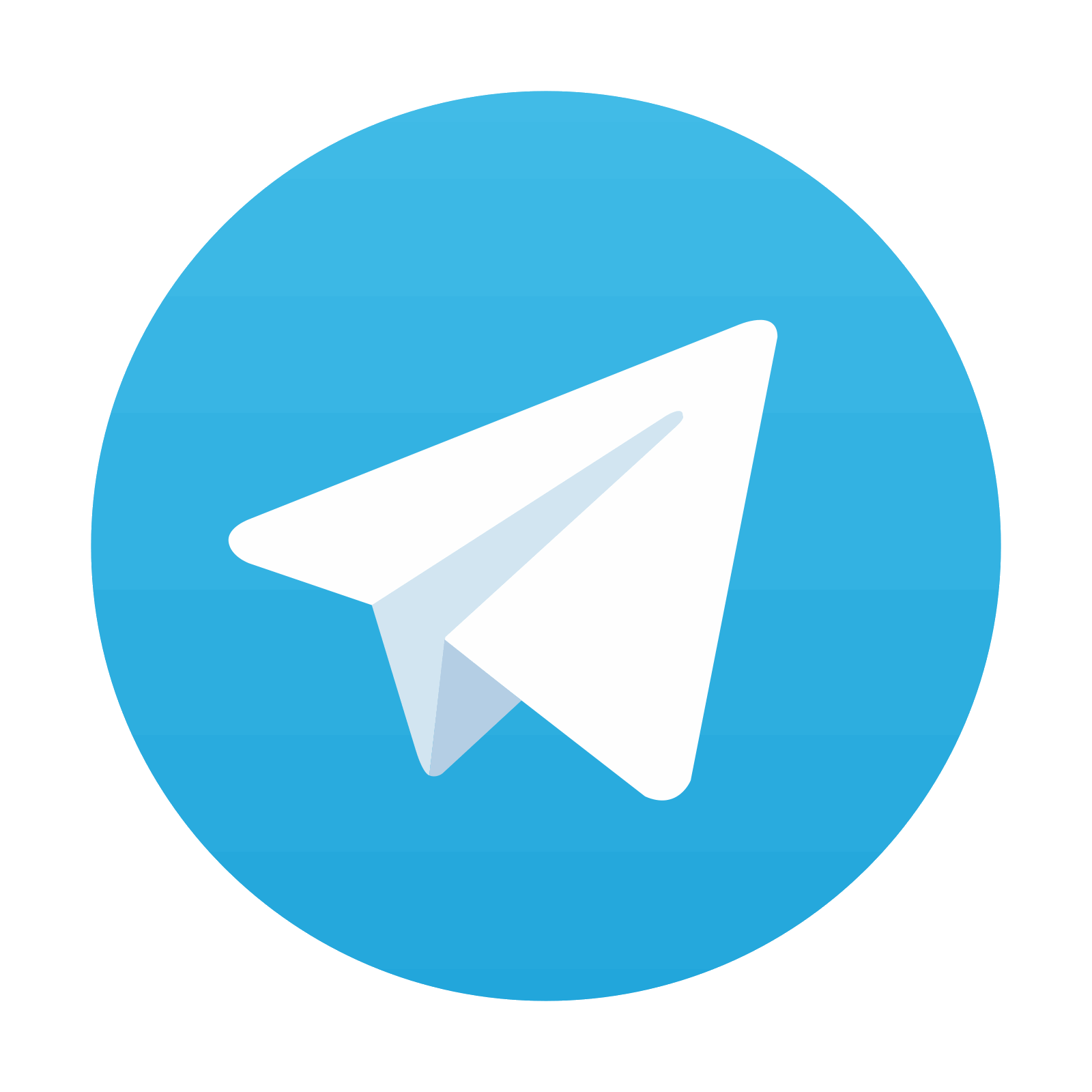
Stay updated, free articles. Join our Telegram channel
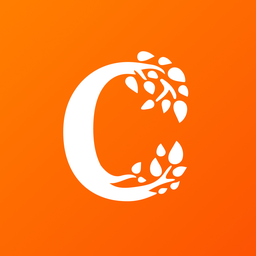
Full access? Get Clinical Tree
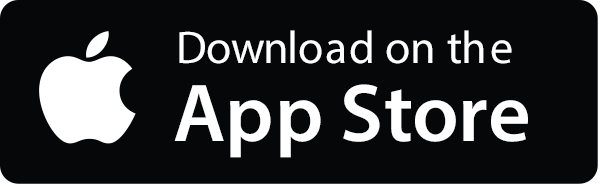
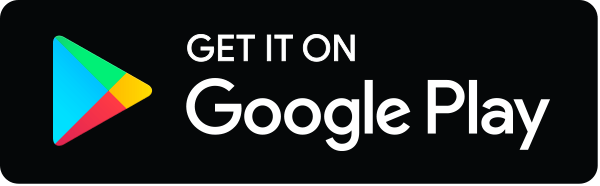