Abstract
Amyotrophic lateral sclerosis (ALS) is a progressive, fatal, neurodegenerative disorder whose pathological hallmark is the loss of motor neurons of the corticospinal pathway. While ALS is a heterogeneous disorder, numerous mechanistic themes, including impaired RNA processing, protein aggregation, intracellular stress, and gliosis, are common to many forms of ALS. In this review we discuss how these mechanisms contribute to selective motor neuron vulnerability and degeneration. In doing so, we synthesize knowledge gained from genetic, molecular, and biochemical studies of human ALS patients and ALS model systems. We conclude with a systematic discussion of the interplay of disease mechanisms and opportunities these mechanisms afford for therapeutic intervention.
Keywords
ALS, motor neuron, astrocyte, microglia, RNA binding protein, stress, protein aggregation
Outline
Introduction 62
RNA Metabolism 63
Evidence for RNA Binding Proteins in the Disease Process 63
Altered RNA Splicing, Transport, and Translation 64
Adenosine Deaminase Acting on RNA 2 66
MicroRNAs 66
Protein Aggregation and Toxicity 67
Superoxide Dismutase 1 69
TAR DNA-Binding Protein-43 70
Fused in Sarcoma 71
Other Aggregating Proteins 72
C9ORF72 72
Axonopathy and Axonal Transport Defects 74
Cellular Stress 76
Stress Granules 76
Autophagy and Proteasomal Stress 77
Endoplasmic Reticulum Stress 79
Inflammation and Glial Function 80
Astrocytes 82
Microglia 83
Oligodendrocytes 84
T Cells 85
Perineuronal Nets and Extracellular Matrix Dysfunction 86
Conclusions 87
References
Introduction
Amyotrophic lateral sclerosis (ALS) is recognized as the most common form of adult-onset motor neuron disease. This progressive, fatal neurodegenerative disorder occurs in approximately two persons per 100,000. Since the initial description of the symptoms and associated pathology in 1874, considerable insights into the genetic, molecular, and biochemical mechanisms of ALS have been gained. The pathological hallmark of ALS is the death of pyramidal motor neurons of the corticospinal pathway in the motor cortex and spinal column. This leads to a myriad of clinical symptoms, such as muscle weakness, muscle atrophy, and spasticity. Considerable variability in site of onset, rate of progression, and survival time occurs in ALS patients and underscores the overall heterogeneity of the disease, which can result from multiple etiologies. This suggests that ALS may represent a collection of disorders that produce similar pathological and clinical phenotypes. The purpose of this chapter is to discuss various mechanisms of ALS, understand how these mechanisms contribute to selective motor neuron vulnerability/degeneration, discuss mechanistic insights gained from ALS model systems, and develop a systematic view of how these mechanisms converge to produce the disease phenotype.
Broadly, ALS can be separated into two categories based on etiology. The vast majority of cases are classified as sporadic ALS (SALS) and are of unknown cause. Approximately 5–10% of all ALS cases are the result of inherited genetic abnormalities and are thus classified as familial ALS (FALS). Although FALS cases are a small fraction of the overall ALS population and are further stratified on the basis of the underlying genetic mutation, considerable insight into disease mechanisms have been gained by studying these rare forms of ALS. As we will emphasize throughout this review, FALS and FALS model systems have proven highly valuable for the study of both FALS and SALS. These monogenic disease-causing variants provide an approach to understand how a triggering event (in this case a genetic abnormality) can produce a cascade of molecular events that ultimately lead to motor neuron degeneration. Our approach is thus to discuss individual mechanisms associated with ALS, combining evidence from research on SALS, FALS, and ALS model systems. This overview will serve as groundwork for building a systematic framework that examines how the interplay of various mechanisms lead to ALS and opportunities these mechanisms present for therapeutic intervention.
RNA Metabolism
Evidence for RNA Binding Proteins in the Disease Process
Since the initial discovery of the TAR DNA-binding protein (TDP-43) as a major component of neuronal cytoplasmic inclusions in ALS and subsequent identification of genetic alterations in the TARDBP gene that cause familial forms of ALS and frontotemporal dementia (FTD), the number of RNA/DNA-binding proteins associated with ALS has expanded considerably ( Table 4.1 ). TDP-43-positive neuronal inclusions are a pathologic hallmark of both ALS and FTD. Mutations in TARDBP account for approximately 4% of familial cases and a small number of apparently SALS cases.
Gene | Locus | Protein | References |
---|---|---|---|
TARDBP | 1p36.22 | TDP-43 | |
FUS | 16p11.2 | Fus | |
SETX | 9q34.13 | Senataxin | |
ANG | 14q11.1 | Angiogenin | |
TAF15 | 17q11.1–q11.2 | TAF15 | |
ATXN2 | 12q23–q24.1 | Ataxin-2 | |
EWSR1 | 22q12.2 | EWSR1 | |
ELP3 | 8p21.1 | ELP3 | |
hnRNPA1 | 12q13.1 | hnRNPA1 | |
hnRNPA2B1 | 7p15.2 | hnRNPA2B1 | |
RBM45 | 2p31.2 | RBM45 |
Shortly after the discovery of TARDBP mutations that cause ALS, missense mutations in the fused in sarcoma (FUS) gene were identified as the cause of chromosome-16p-linked FALS. Mutations in FUS also account for ~4% of FALS cases. The observed protein amino acid domain homology between TDP-43 and FUS, with both proteins containing multiple RNA binding motifs, suggested that RNA metabolism may play an important role in ALS. Other key structural elements include the presence of glycine-rich domains and prion-like domains that contribute to their pathological aggregation and impaired function in ALS. This latter element was used to predict other RNA binding proteins associated with ALS. This led to the discovery of disease-causing mutations in TAF15 , hnRNPA1 , and hnRNPA2B1 . Key functional properties linking these RNA binding proteins include association with stress granules (SGs) and nucleocytoplasmic translocation during cellular stress.
Genetic studies have identified other RNA binding proteins linked to familial or sporadic forms of ALS. These include disease-causing mutations in SETX , ANG , and ELP3 , repeat expansion of ATXN2 associated with increased risk of ALS, and others such as RBM45 that are linked to ALS due to pathologic inclusions of the protein that occur in patients.
Altered RNA Splicing, Transport, and Translation
While most of the genetic alterations of RNA binding proteins impact their subcellular distribution and accumulation into SGs and/or pathologic inclusions (see below), we will focus here on specific effects of disease-causing mutations in TDP-43 and FUS on RNA metabolism ( Fig. 4.1 ). RNA binding proteins have diverse roles in the cell and function within many nuclear substructures and the cytoplasm. At present, the vast majority of evidence for impaired RNA processing in ALS has come from studies of TDP-43 and FUS. However, many other RNA binding proteins linked to ALS interact with TDP-43 and/or FUS, and therefore likely impact RNA metabolism. Since both TDP-43 and FUS bind RNA/DNA ( Fig. 4.1 ), determining the specific binding sequences and effects on gene expression were crucial to understanding how these proteins contribute to cell death in ALS. Using CLIP-SEQ, TDP-43 was shown to bind over 6000 RNA targets in the brain, approximately 30% of the transcriptome. TDP-43 binding to long introns (>100 kb) is required for the normal maturation and splice site selection of immature mRNA species. TDP-43 binding to the 3′-UTR of mRNAs may impact on stability or transport, whereas binding to long noncoding RNAs (ncRNAs) may influence their regulatory roles. Splicing of many RNA targets of TDP-43 is altered in ALS spinal cord tissue.

Likewise, FUS binds over 5500 RNA targets in the brain, and its binding pattern to long introns suggests that FUS remains bound to pre-mRNAs until splicing is complete. Loss of FUS function results in changes of the splicing pattern or abundance in over 1000 RNAs. All three members of the FET gene family (FUS, EWSR1, and TAF-15) are implicated in ALS, suggesting that the functions of these proteins on global RNA splicing and metabolism are particularly important for motor neurons. In addition, both TDP-43 and FUS bind to long ncRNAs (lncRNA) and influence their function and subcellular localization ( Fig. 4.1 ). Both proteins, e.g., bind NEAT1, a lncRNA core component of nuclear paraspeckles, which function in cell stress responses and in the nuclear retention of hyperedited RNAs. FUS directly regulates NEAT1 levels and decreasing FUS levels leads to reduced numbers of paraspeckles. At the same time, FUS-positive inclusions contain other paraspeckle proteins, suggesting that pathological changes in FUS levels or function impair normal paraspeckle formation/function, thereby altering cellular homeostatic responses and increasing motor neuron vulnerability to degeneration.
Finally, both TDP-43 and FUS exhibit neuron-specific functions that further implicate them in neurodegenerative diseases. Both localize to dendrites in response to neuronal activity. Disease-causing mutations that result in functional impairments and protein mis-localization therefore likely impact on synaptic structure and function via loss of localized translation of specific mRNAs. Given the remarkable functional diversity of RNA binding proteins such as TDP-43 and FUS, it is unsurprising that mutations that affect their structure and function confer numerous aberrant changes in the regulation of gene expression. Motor neurons, in particular, seem acutely vulnerable to alterations in the levels of ALS-linked RNA binding proteins by either loss of function, gain of toxic function, or both. While much insight into the sequence targets, processing functions, and subcellular associations of ALS-associated RNA binding proteins have been gained in recent years, determining which of these properties contribute directly to motor neuron vulnerability/degeneration remains an unanswered question with considerable therapeutic implications.
Adenosine Deaminase Acting on RNA 2
Further evidence for motor neuron-specific defects in RNA processing have come from studies of RNA editing of the GluA2 subunit of the l-α-amino-3-hydroxy-5-methyl-4-isoxazolepropionic acid (AMPA) receptor. Adenosine deaminase acting on RNA 2 (ADAR2)-mediated conversion of adenosine to inosine (A-to-I editing) of the GluA2 pre-mRNA results in replacement of glutamine with arginine in the translated protein. This editing normally occurs in all motor neurons and results in expression of Ca 2+ -impermeable AMPA receptors. ADAR2 levels, and consequently A-to-I editing, are dramatically reduced in SALS motor neurons. The resultant enhancement of AMPA Ca 2+ permeability leads to increased motor neuron vulnerability and the development of TDP-43 pathology.
MicroRNAs
Over the past decade, microRNAs (miRNAs) have been identified as significantly impacting overall gene expression by modulating the stability and/or translational repression of target mRNAs. miRNAs are short noncoding RNAs (~22 nucleotides) and over 1000 have been identified in humans, constituting a large class of regulators of gene expression. Approximately 60% of all mammalian mRNAs are predicted targets of miRNAs. miRNA binding to mRNA targets results in reduced protein expression from the specific bound mRNA. A single miRNA may have many mRNA transcript targets, therefore impacting on the expression of a large number of genes. Various types of cellular stress impact on the levels of miRNAs and therefore regulate how a cell responds to stress. As we further describe below, stress responses during ALS represent an important pathogenic mechanism and therefore miRNAs likely contribute to the overall ability of a cell to properly respond to acute or chronic stress conditions that exist during ALS.
Recent studies have examined miRNA changes in ALS patients and model systems, including the G93A SOD1 transgenic mouse model, circulating monocytes, skeletal muscle, and lumbar spinal cord tissue from ALS patients, and serum from FALS patients and premanifest carriers of genetic mutations. Altered levels of miRNAs may have significant impact on gene expression during ALS ( Fig. 4.2 ). Perhaps due to differences in models, cell/tissue types, and analytical methods used in these studies, a common set of miRNA alterations was not observed. However, two studies detected increased levels of miR-146a and miR-155 in microglial cells. In addition, increases in miR-146a were detected in two studies using either peripheral monocytes or spinal cord tissue from ALS patients. The results support further studies on miRNA changes in ALS using standardized approaches and model systems, ideally in large collaborative research efforts. Pathway analysis of genes modulated by the miRNAs altered in ALS will provide important information regarding mechanistic pathways regulated by miRNAs during ALS and possibly very early changes in these pathways. In addition, miRNAs may correlate with the rate of ALS disease progression, as miR-206 levels have been associated with the rate of disease progression in the G93A SOD1 mouse model. Finally, both TDP-43 and FUS (and likely other RNA binding proteins implicated in ALS) impact miRNA biogenesis, linking ALS disease-causing mutations to miRNA-regulated gene expression.

Protein Aggregation and Toxicity
The abnormal aggregation of proteins into inclusion bodies in motor neurons is a well-known pathological feature of both SALS and FALS. Early characterizations of inclusions defined their filamentous, skein-like morphology, along with their eosinophilic core (and, hence, proteinaceous composition). Subsequent work revealed a variety of characteristic inclusion types in ALS motor neurons, including larger skein-like inclusions reactive for ubiquitin, smaller filamentous inclusions containing neurofilament proteins, dense spheroids with a Lewy body-like appearance (compact inclusions), and Bunina bodies, small granular inclusions of lysosomal origin. Since the identification of inclusions as a pathological hallmark of ALS, considerable effort has been devoted to determining the protein constituents and neurotoxic mechanisms.
Superoxide Dismutase 1
The discovery that point mutations in the superoxide dismutase 1 ( SOD1 ) gene can produce familial forms of ALS (approximately 20% of all FALS cases ) led to the identification of mutant, misfolded SOD1 protein within inclusions. Subsequent work has shown that SOD1 pathology can also occur in SALS cases. SOD1 catalyzes the conversion of the superoxide anion into hydrogen peroxide and molecular oxygen via the cyclical reduction and oxidation of copper. Despite altered enzymatic activity in some FALS-linked SOD1 mutant proteins, existing evidence suggests that loss of SOD1 antioxidant function does not contribute to SOD1-linked FALS. Notably, disease progression and severity are not correlated with mutant SOD1 enzymatic activity in human patients and mice lacking the SOD1 gene develop normally and do not develop motor deficits, in contrast to mutant-SOD1-expressing mice that develop progressive motor abnormalities and SOD1 aggregates. FALS-linked mutant SOD1 aggregates into insoluble amyloid-like fibrils in vitro, in transgenic mutant-SOD1-expressing mice, and in FALS patients. The mutant SOD1 aggregation is driven by mutation-induced misfolding, which can result from the protein sequence itself, reduced capacity to bind metal ions, or both.
While human ALS patients and transgenic G93A SOD1 mice develop insoluble, ubiquitinated SOD1 aggregates in motor neurons, current models implicate soluble, misfolded SOD1 as the toxic species, similar to models proposed in other aggregating proteins, such as amyloid beta. Soluble, misfolded SOD1 can form oligomeric pore structures and exerts many deleterious effects within the cell. The protein is capable of inducing endoplasmic reticulum (ER) stress, which overwhelms the cell’s capacity to provide normal clearance of cytoplasmic proteins and can ultimately lead to apoptotic cell death. Mutant SOD1 also aberrantly accumulates in the mitochondrial intermembrane space, impairing normal mitochondrial function. Where the mutation reduces metal ion binding, mutant SOD1 also disrupts calcium homeostasis, enhancing susceptibility to cellular stress. More recent work suggests that cells also secrete misfolded SOD1, which can then seed the aggregation SOD1 (mutant or wild-type native) in adjacent cells via a prion-like process, providing a possible mechanism for spread of disease. Therefore, mutant SOD1 may induce intracellular mechanisms of cell death and propagate spread of disease via cell–cell communication pathways.
TAR DNA-binding protein-43
Subsequent work demonstrated that many ubiquitinated, skein-like inclusions in SALS motor neurons were nonreactive to anti-SOD1 antibodies. The identity of the constituent protein(s) of these inclusions remained elusive until 2006, when TDP-43 was identified as the main component of cytoplasmic inclusions in ALS. Subsequent studies identified mutations in the TARDBP gene that induce familial forms of either ALS or frontotemporal lobar dementia (FTLD), each with extensive TDP-43 inclusion pathology. These disorders show a number of common TDP-43 modifications, the most prevalent of which is the incorporation of the protein into insoluble, ubiquitinated filamentous inclusions in neurons. The protein is also frequently depleted from the cell nucleus, hyperphosphorylated, and cleaved into C-terminal fragments (CTFs), though this latter phenomenon occurs in a central nervous system (CNS) region-specific pattern.
TDP-43 contains two RNA recognition motifs (RRMs), a nuclear localization sequence (NLS), a nuclear export signal, a glycine-rich C-terminal domain, and a prion-like domain, the latter two of which are aggregation prone and the location of the majority of ALS/FTLD-causing TARDBP mutations. As described above, TDP-43 has many roles in the regulation of gene expression. The importance of this regulatory capacity is underscored by the observation that TARDBP null mice are embryonic lethal. TDP-43 also undergoes altered nucleocytoplasmic transport in response to cellular stress. The resultant association of the protein with cytoplasmic SGs, stress-induced protein–RNA complexes containing stalled translational components ( Fig. 4.2 ), has emerged as a plausible mechanism for TDP-43 aggregate formation.
Unlike SOD1, TDP-43 toxicity is mediated by both loss of function and toxic gain of function mechanisms. Loss of the normal gene expression regulatory functions of TDP-43 may occur through hypofunction induced by mutation or posttranslational modification (e.g., phosphorylation or C-terminal cleavage). Similarly, trapping of the protein in cytoplasmic aggregates is expected to contribute to loss of function by depleting the protein from the nucleus and impairing both normal nuclear and cytoplasmic TDP-43 functions. The importance of maintaining homeostatic TDP-43 levels to cell viability is highlighted by studies demonstrating TDP-43’s ability to self-regulate its expression in cells via an auto-regulatory feedback loop and the observation of neuronal and behavioral abnormalities in a variety of model systems over- or underexpressing TDP-43 (wild-type or mutant), including mice, Drosophila, and zebrafish.
In addition, misfolded, aberrantly modified TDP-43 displays toxic properties unrelated to its normal functions that are primarily related to the cytoplasmic accumulation and processing of the protein. Increased cytoplasmic accumulation is an early event in human TDP-43 proteinopathies and is thought to precede inclusion formation. When recapitulated in cell culture and animal models, the phenomenon induces TDP-43 aggregation/misfolding, proteasomal and autophagic activation, and is toxic to cultured neurons. Cellular stress together with aberrant cytoplasmic localization of TDP-43 promotes cleavage of the protein into CTFs by the activation of caspases. The precise function(s) of the fragments remains elusive; however, CTFs are hyperphosphorylated and toxic to cultured cells. Likewise, the effects of TDP-43 phosphorylation are not entirely clear, though these modifications are strongly associated with misfolded, insoluble forms of the protein. Misfolded, cytoplasmic TDP-43 also appears capable of seeding further misfolding of endogenous TDP-43 in a prion-like manner, as observed for SOD1.
Fused in Sarcoma
FUS/translocated in sarcoma is a related RNA binding protein also mutated in rare instances of FALS (approximately 4% of all FALS cases) and found in skein-like inclusions in FALS and SALS motor neurons. Structurally, the protein contains an RRM, an NLS, a nuclear export sequence, two arginine/glycine-rich regions, a zinc finger domain, a prion-like domain, and a glycine-rich domain. These latter two features confer a propensity for aggregation to the protein. Like TDP-43, it has numerous roles in the regulation of gene expression. FUS binding to DNA regulates transcription/splicing and the protein is recruited to sites of DNA damage, where it contributes to break repair. FUS also regulates transcription by direct binding to DNA. FUS also binds long intronic regions of pre-mRNAs and contributes to splicing and the protein also regulates the subcellular localization of RNA molecules. FUS, like TDP-43, is capable of nucleocytoplasmic shuttling by virtue of its structure and, when localized to the cytoplasm, associates with SGs. As it does for TDP-43, this association provides a plausible mechanism for FUS inclusion formation during ALS.
Both loss of function and toxic gain of function mechanisms are thought to contribute to FUS-mediated neurodegeneration. The trapping of FUS in inclusions is expected to impair the normal functions of FUS and neurons appear particularly sensitive to mutations that alter FUS levels or confine the protein to the cytoplasm. Like TDP-43, several ALS-causing FUS mutations are found in the glycine-rich region of the protein, while, in contrast to TDP-43, numerous additional disease-causing mutations have been found in the NLS. This underscores the vital role of intranuclear FUS functions to cell viability and the toxic properties of excessive cytoplasmic FUS. Cytoplasmic-confined FUS forms inclusions and these are positive for ubiquitin and p62. Intriguingly, these same inclusions are negative for TDP-43, implying separate mechanisms of inclusion formation, despite the association of both proteins with SGs. FUS inclusions are nonamyloid, though the protein can form fibrils in vitro. Insoluble FUS can be found in human ALS tissue and FUS inclusions are frequently ubiquitinated. Collectively, these processes suggest that FUS induced neurodegeneration includes altered protein distribution to the cytoplasm, misfolding, and inclusion formation in both FUS-linked FALS and some SALS patients.
Other Aggregating Proteins
In recent years, gene mutations in a number of other aggregation prone proteins have been found in FALS. These include mutations in the UBQLN2 , OPTN , SQSTM1 , VCP , HNRNPA1 , HNRNPA2B1 , and PFN1 genes. Despite diverse functions of each protein, aggregates of the mutant protein are consistently found in FALS cases. Moreover, recent work has shown that non-ATG translation of the FALS-associated C9ORF72 repeat expansion leads to the expression of dipeptide repeat proteins (DPRs) that are aggregated in FALS tissue and model systems. These findings underscore the preponderant role of protein aggregation in motor neuron degeneration, especially when considered together with the observation that similar aggregates of these proteins are frequently found in SALS cases. Moreover, some FALS genes encode proteins associated with the ubiquitin proteasome system, demonstrating the indispensable role of protein quality control and degradation in maintaining neuronal viability. While the protein composition of aggregates in SALS and FALS varies considerably, proteasomal stress and impairment of normal cellular trafficking of biomolecules are expected to occur widely in the various forms the disorder takes and, thus, these processes represent promising therapeutic targets for future drug development and clinical trials.
C9ORF72
The most common genetic cause of ALS and FTLD is a GGGGCC repeat expansion in the C9ORF72 gene. The C9ORF72 repeat expansion is believed to underlie approximately 40% of FALS and 6–7% of SALS cases. Patients with C9ORF72 repeat expansions that develop disease display variable amounts of TDP-43 pathology and abundant TDP-43 negative, p62-positive neuronal and glial inclusions in multiple regions of the CNS, including the cerebellum. At present, the function(s) of the c9ORF72 protein is not fully characterized; however, the protein’s high degree of homology with the DENN protein, a GDP/GTP exchange factor that acts on Rab-GTPases, and reported ability to regulate endosomal trafficking suggest that the protein has multiple functions.
Proposed neurotoxic mechanisms of the repeat expansion include haploinsufficiency and a toxic gain of function. Studies of the former have produced conflicting results: conditional knockout of the c9ORF72 gene does not result in the development of motor neuron degeneration or an overt motor/behavioral phenotype in mice; however, knockdown of the zebrafish ortholog (zc9ORF72) led to motor deficits and axonal pathology. Differences in model system, genetic ablation strategy, and repeat expansion size in these studies could account for these discrepancies and underscore the complexity of C9-mediated neurodegeneration. Experimental evidence for a repeat expansion induced toxic gain of function have implicated both the accumulation of RNA foci within the nucleus and cytoplasmic translation of dipeptide proteins via repeat-associated non-AUG translation (RAN) translation products and resultant accumulation of DPR cytoplasmic inclusions. The presence of sense and antisense foci is a common observation in both patient tissues/cell lines and C9 model systems, and C9 transcripts are capable of forming G-quadruplex structures that may aberrantly influence gene expression by sequestering RNA binding proteins. As noted above, c9ORF72 FALS patients exhibit DPR cytoplasmic inclusions resulting from non-ATG (RAN) translation of the repeat. DPRs are neurotoxic in a variety of ALS model systems and coculture of astrocytes taken from C9 patients with wild-type motor neurons results in extensive neuronal death, suggesting intracellular and noncell autonomous mechanisms of neurodegeneration in C9-linked ALS. Studies in ALS patients, however, have noted the presence of DPR-containing inclusions in regions of the CNS that do not degenerate in ALS, suggesting that DPR inclusions are not directly neurotoxic.
Recent studies examining mechanisms of C9-mediated toxicity have consistently identified impairments in nucleocytoplasmic transport of proteins. It remains unclear whether all proteins that enter/exit the nucleus are equally impacted by C9 repeat expansion induced transport defects. Therapeutic approaches that enhance nucleocytoplasmic transport or remedy defective transport-induced pathologies may thus be an effective treatment for C9-linked ALS. However, nucleocytoplasmic transport deficits may be present from birth, whereas ALS or FTLD typically occurs in late to middle age, raising the question of whether additional factors cause disease and C9 repeat expansion induced nucleocytoplasmic transport defects merely make the cell more susceptible to further insult. Antisense oligonucleotides (ASOs) and small molecules that target production of C9 RAN proteins have also been identified as therapeutics with clinical potential. ASOs have been shown to ameliorate or prevent the development of C9-associated pathology and pathophysiology in some model systems. However, since C9 repeat expansion BAC transgenic mice harbor both nuclear RNA foci and C9 RAN proteins throughout life yet exhibit no phenotype, the role of both RNA foci and C9 RAN protein inclusions in ALS neurodegeneration remains uncertain. Moreover, while loss of C9ORF72 function does not induce motor neuron degeneration or impair CNS function, the necessity of the c9ORF72 protein for the proper functioning of other organ systems/cell types has not been evaluated. Thus, while knockdown-based approaches (such as ASOs) have shown early promise in model systems, much further research is needed to determine their safety/efficacy.
Axonopathy and Axonal Transport Defects
Synaptic activity at the level of the axon is energetically demanding and requires a diverse array of biochemical reactions, cellular organelles, and molecules for function. Anterograde axonal transport facilitates these processes by transporting proteins, mRNAs, organelles, vesicles, and other signaling molecules to the axon terminal. At the same time, the axon requires a means to retrogradely transport misfolded proteins, damaged organelles, vesicles, and signaling molecules from the axon to the cell soma. These functions are accomplished by axonal transport using protein molecular motor complexes in conjunction with the axonal cytoskeleton. Because motor neuron axons may be up to 1 m in length, they are particularly vulnerable to impairments in axonal transport and defects in cytoskeletal structure.
The pathological aggregation of cytoskeletal intermediate filament proteins is a common pathological observation in SALS and FALS. Spheroidal axonal aggregates, Lewy body-like inclusions, and hyaline conglomerate inclusions are frequently positive for peripherin and the neurofilament proteins in FALS and SALS cases. These proteins are critical components of the neuronal cytoskeleton and modulate intracellular transport. Peripherin forms cytoskeleton-associated homopolymers that can also interact with other cytoskeletal proteins. These cytoskeletal intermediate filament proteins are largely localized to the axon where they provide structural strength and regulate axonal diameter. The neurofilament cytoskeletal assembly is highly dynamic and the rate of transport of neurofilament proteins is inversely related to their phosphorylation state. The abnormal accumulation and aggregation of these intermediate filament proteins occur in both the cell body and the axon of ALS motor neurons. In the case of neurofilaments, the aggregated proteins are generally hyperphosphorylated, and hence, immobile, suggesting impaired cytoskeletal dynamics. Genetic evidence for motor neuron vulnerability to impaired cytoskeletal structure comes from the identification of rare mutations in the peripherin or neurofilament heavy chain genes in ALS patients, and the observation that peripherin-overexpressing mice develop intermediate filament protein inclusions and progressive motor neuron loss.
The remarkable length of motor neuron axons and the necessity of axonal transport for synaptic function implicates axonal transport as a susceptibility factor in motor neuron degeneration. A dying-back mechanism was initially suggested by the identification of decreased retrograde transport in motor neuron axons from ALS patients. Impairments in axonal transport were further implicated as a susceptibility factor for motor neuron degeneration when mice overexpressing the human neurofilament heavy protein were found to develop selective, progressive motor neuron degeneration. Mice overexpressing dynamitin showed deficits in retrograde axonal transport related to the disassembly of the dynactin complex and motor neuron degeneration. Thus, impairment of axonal transport in either direction is sufficient to cause motor neuron degeneration.
Transgenic mice expressing G93A SOD1 also demonstrate that impaired axonal transport contribute to motor neuron degeneration. Impairments in anterograde axonal transport is one of the earliest pathologic events that occurs prior to symptom onset. Slowed anterograde transport in these mice, particularly of tubulin and neurofilament proteins, leads to axonal swellings containing these proteins. Other studies have suggested that impairments in fast axonal transport leads to depletion of axonal mitochondria, due to intact retrograde transport. However, others have implicated reduced retrograde transport in transgenic SOD1 mice by virtue of the fact that mutant SOD1, but not wild-type protein, interacts directly with the dynein complex. Intriguingly, expression of mutant SOD1 alters not simply the rate of retrograde transport, but the cargo as well. The dynein-associated fraction of axoplasm from mutant SOD1 transgenic mice shows a significant reduction of neurotrophic factors (including nerve growth factor and brain-derived neurotrophic factor) and significant increases in stress-associated signaling proteins (including caspase-8 and pJNK). Despite apparent discrepancies, these studies all indicate that impaired axonal transport is an early, presymptomatic event in transgenic SOD1 mice.
Recent work has shown that TDP-43 also associates with mRNAs in the cytoplasm and is transported via slow axonal transport. FALS-linked mutant TDP-43 misfolds and reduces the net movement of mRNA granules and shifts the balance of axonal transport in the retrograde direction. Thus, impaired axonal transport and cytoskeletal abnormalities at the level of the axon are susceptibility factors for motor neuron degeneration in various forms of ALS. The loss of normal cytoskeletal structure and trafficking of cargoes impairs neuronal function, while protein aggregates and enhanced stress signaling are associated with neuronal toxicity. Defects in axonal transport are consistently an early event observed in ALS model systems, suggesting it represents an early point for therapeutic intervention. Altered nucleocytoplasmic transport in patient with the C9 repeat expansion may disrupt axonal transport and lead to cognitive deficits in FTD due to axonopathy in cortical brain regions.
Cellular Stress
Intracellular stress occurring in motor neurons is widely recognized as a molecular mechanism leading to ALS pathology and motor neuron death. Studies in human ALS tissues and model systems have documented the occurrence of multiple forms of intracellular stress occurring within motor neurons and the ways these stressors may contribute to cell death. Though it is likely that multiple cellular stresses contribute to cell death in concert, understanding the effects of a single cellular stressor is valuable in defining the time course of events that lead to cell death and in identifying potential therapeutic targets.
Stress Granules
Cytoplasmic SGs are protein–RNA complexes that form in response to a variety of cellular stressors. The onset of cellular stress causes a rapid cessation of mRNA translation. The stalled translational complex rapidly disassembles and the components coalesce into SGs. The purpose of SGs appears to be two-fold: (1) to prioritize translation of mRNAs that favor the cellular response to stress, and (2) to remove other mRNAs and RNA binding proteins from the harmful cellular environment and stop their translation. SGs are dynamic, transient structures that dissipate following the removal of the stressor and a constant turnover of protein and mRNA occurs during the life of the SG. Multiple lines of evidence now suggest SG formation and duration are vital determinants of cell fate decisions following stressor onset or in disease.
The notion that this adaptive response has a role in ALS pathogenesis has roots in two discoveries: (1) that TDP-43 is a primary component of ubiquitinated inclusions in ALS/FTLD patients, and (2) that TDP-43 translocates from the nucleus and associates with SGs during conditions of cellular stress. Subsequent identification of FUS as a component of inclusions, FUS mutations leading to FALS, and its association with SGs furthered the notion that SGs represent potential sites of cytoplasmic inclusion formation. Analysis of the domain structure of TDP-43 and FUS (and other SG-associated proteins) provided evidence of the structural elements mediating the association of these proteins with SGs, as well as the mechanisms by which these proteins may be irreversibly converted from a transient SG assembly into insoluble aggregates. TDP-43 incorporation into SGs requires RRM1 and the glycine-rich C-terminal component of the protein, which is aggregation prone. For FUS, the protein’s zinc finger RNA binding domain is required and the degree of association is mediated by the protein’s RRM and glycine-rich domain. The necessity of intact RNA binding for incorporation of both proteins suggests that their association with SGs is part of the normal cellular response to stress and that loss of this function adversely affects motor neuron viability.
Though the aggregation of proteins into fibrils can serve beneficial functions in yeast in certain environments, the persistent association of TDP-43, FUS, and other RNA binding proteins in SGs in ALS is thought to result in the generation of toxic oligomers and fibrils and the loss of DNA/RNA binding functions by misfolding and removal of these proteins from their normal cellular milieu. Moreover, emerging evidence suggests that a host of RNA binding proteins may participate in this process. Analysis of prion-like domain harboring proteins in the human proteome shows a striking enrichment of DNA/RNA binding proteins, with as many as 20% of proteins with prion-like, low complexity domains having RNA binding function. The identification of mutations in the genes encoding these proteins (e.g., TARDP , HNRNPA1 , and others ) in FALS cases and the association of these proteins with SGs provides additional evidence in support of this concept. Thus, SGs contribute to ALS pathogenesis by both toxic loss of normal function and toxic gain of function and may thus be a point of therapeutic intervention.
Autophagy and Proteasomal Stress
Autophagy is a degradative pathway by which intracellular material, including proteins and organelles, are enveloped and degraded via fusion with lysosomes. The function of autophagy and the observation of numerous types of proteinaceous inclusions in ALS suggests a role for autophagy in the disease process. Many studies consistently find autophagy defects in human ALS tissue and ALS model systems. While many signaling molecules mediate various steps in autophagy, among the more well-characterized are phosphatidylinositol 3-kinase signaling during autophagosome formation and p62/SQSTM1 tagging of proteins/structures targeted for degradation. Autophagy is also crucial for the clearance of misfolded or aggregated proteins and is activated by the unfolded protein response (UPR). The protein targets of autophagy appear to be relatively long-lived proteins, which includes RNA binding proteins, in contrast with the ubiquitin/proteasome system, which is relatively specific for short-lived proteins.
Autophagic clearance of proteins and organelles is particularly important for neuronal homeostasis and survival, as neurons have considerable metabolic needs and are acutely sensitive to the presence of misfolded or aggregated proteins. ALS patients show accumulation of p62-positive inclusions in motor neurons and glia, suggesting insufficient clearance of misfolded and aggregated proteins. The identification of mutations in genes encoding autophagy-associated proteins as causative factors in subsets of FALS population strengthened the proposed role of autophagy in motor neuron homeostasis ( Fig. 4.2 ). Mutations in OPTN , the gene encoding optineurin, a membrane trafficking protein and autophagy receptor for damaged mitochondria, cause rare forms of FALS. OPTN -linked FALS cases harbor optineurin-positive inclusions, which also contain TDP-43, p62, and ubiquitin. Optineurin-positive inclusions are relatively rare in SALS cases.
Mutations in the valosin containing protein (VCP) gene, which encodes valosin-containing protein, are also found in a subset of FALS patients. VCP protein is essential for autophagy and loss of VCP function (either via expression of a nonfunctional mutant protein or gene silencing) leads to the accumulation of unfused autophagosomes and neurodegeneration. Intriguingly, OPTN and/or VCP mutations also lead to the accumulation of cytoplasmic TDP-43 inclusions, suggesting that autophagic hypofunction is sufficient to promote TDP-43 nuclear translocation, aggregation, and ultimately, cell death. Therefore, SALS and FALS are united by the common motor neuron susceptibility factor of impaired or overwhelmed autophagic function and TDP-43 aggregation. VCP protein is not incorporated into cytoplasmic inclusions in ALS patients or model systems, further suggesting that loss of autophagic function is the primary pathogenic mechanism of VCP mutations. Finally, FALS-causing mutations in genes for the autophagy receptor protein p62 ( SQSTM1 ), UPS and autophagy-associated protein ubiquilin 1 ( UBQLN1 ), and the endosomal trafficking component charged multivesicular protein 2B ( CHMP2B ) provide further evidence that impaired protein/organelle clearance and vesicular trafficking contribute to motor neuron death in ALS patients and model systems.
Activation of autophagy via rapamycin reduces TDP-43 aggregation and ameliorates motor deficits in both transgenic mice expressing TDP-43 and Drosophila overexpressing the TDP-43 ortholog dTDP, suggesting that autophagic balance is a key determinant of motor neuron health and survival in ALS model systems. These results suggest that autophagy activation is a potential ALS therapeutic target. However, administration of rapamycin to SOD1-G93A mice accelerated motor neuron degeneration, suggesting that some caution is necessary in targeting autophagy as a therapy and that autophagic balance is a key determinant of motor neuron health.
Endoplasmic Reticulum Stress
The ER is an integral component in the synthesis, folding, modification, and transport of proteins through the secretory pathway. Perturbation of normal ER function and, particularly, the accumulation of un- or misfolded proteins causes ER stress. ER stress signals that the normal capacity of the ER to modify and fold proteins has been saturated, which triggers the UPR. Key components of the UPR include a general decrease in the rate of protein synthesis, an increase in the expression of ER-associated chaperone proteins, and activation of the endoplasmic reticulum-associated (ERAD) protein degradation pathway and ER-induced autophagy. ERAD initiates translocation of misfolded protein into the cytoplasm for polyubiquitin tagging and proteasomal degradation.
ER function and stress are monitored by three ER membrane proteins with signaling functions: IRE1, PERK, and ATF6. The function of the latter two proteins is regulated by the ER chaperone BiP, which is normally bound to the luminal domains of PERK and ATF6. The accumulation of misfolded proteins releases BiP and allows cytosolic translocation of active PERK and ATF6. PERK inhibits translation (reducing the folding load on the ER) by phosphorylating its target, eIF2α. ATF6 is proteolytically processed to a transcriptional activating form and translocates to the nucleus where it increases the transcription of ER-folding-associated genes. IRE1 is activated by the direct binding of misfolded proteins and also undergoes cytosolic translocation. Once in the cytosol, its RNAse domain leads to removal of an intron in the XBP1 transcript, leading to production of the associated protein, which is a transcriptional activator of ER-fold-associated genes. The ER is also a major reservoir for intracellular Ca 2+ and contains numerous calcium channels and sensors. When UPR and ERAD are insufficient to restore ER homeostasis, the above ER proteins and Ca 2+ signaling can induce apoptosis.
Accumulating evidence suggests that impaired UPR and excessive ER stress are contributing factors to motor neuron vulnerability in ALS. ER abnormalities are common in SALS motor neurons, with irregularities in ER structure, chromatolysis of ER membrane, and ribosomal detachment from ER membrane evident. Spinal cord motor neurons of SALS patients show several signs of ER stress, including enhanced PERK-induced eIF2α phosphorylation and increased expression of ER chaperone proteins. Further evidence that ER homeostasis and UPR are components of selective motor neuron vulnerability in ALS comes from longitudinal studies of vulnerable and resistant motor neurons in G93A SOD1 transgenic mice. Vulnerable motor neurons were consistently more susceptible to the onset of ER stress and showed upregulation of UPR markers compared to resistant motor neurons, despite comparable accumulation of ubiquitinated cytosolic proteins. Cytoplasmic SOD1 inclusions in G93A SOD1 mice are also positive for ER-resident chaperone proteins, suggesting that misfolded SOD1 accumulates in the ER, activates the UPR, and may ultimately overwhelm its capacity, resulting in apoptotic cell death. This, together with evidence that the ER stress-reducing drug salubrinal preserves neuromuscular function and increases survival in SOD1 transgenic mice, argues that the role of ER stress and UPR in disease is complex. Maintenance of ER homeostasis is dependent on multiple sensors and signaling pathways and the relative balance of these, together with other processes like proteasomal and autophagic protein degradation, likely determines whether UPR activation is beneficial or harmful to motor neurons in ALS.
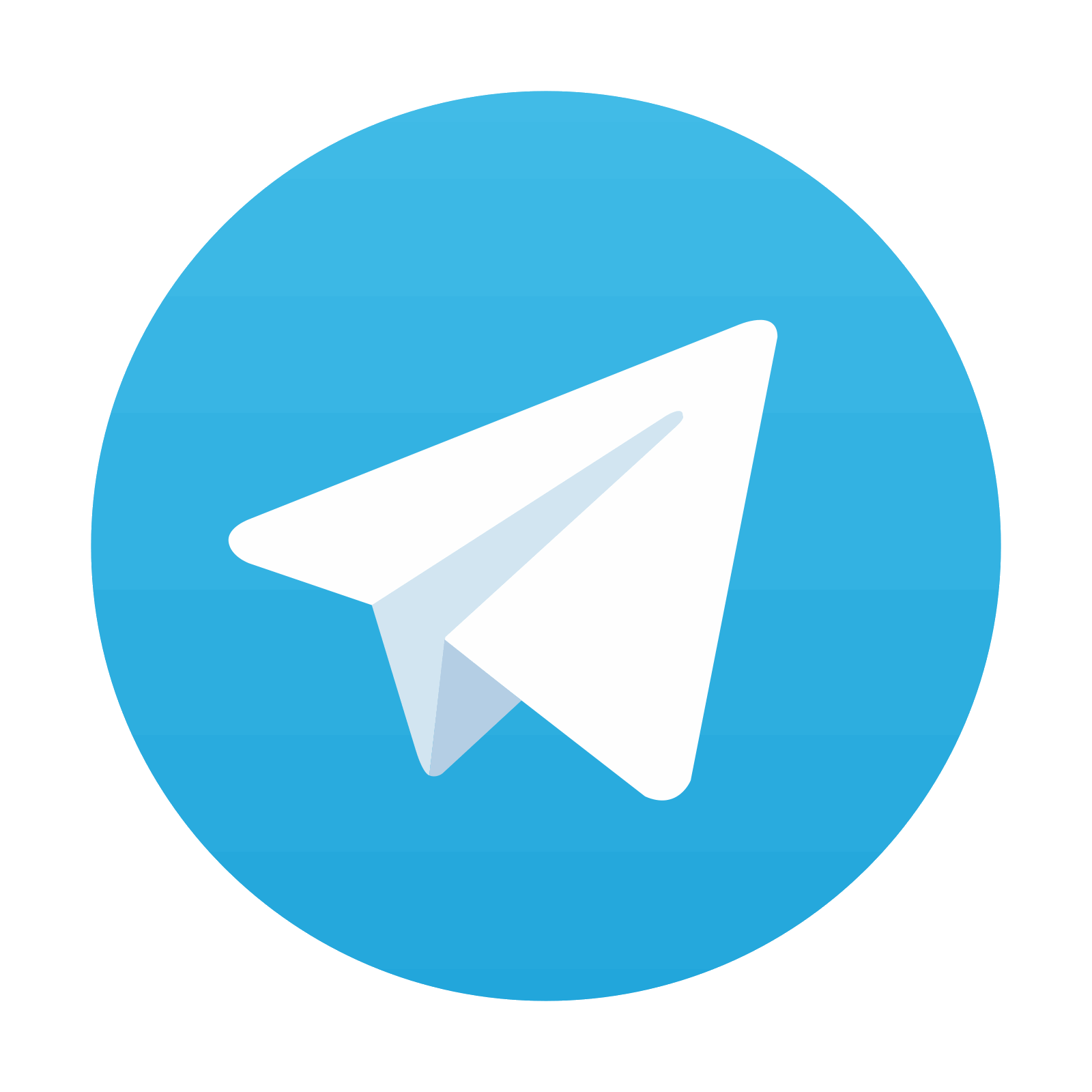
Stay updated, free articles. Join our Telegram channel
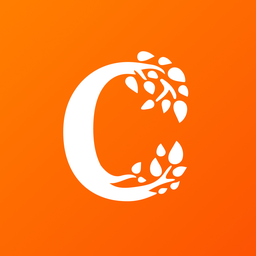
Full access? Get Clinical Tree
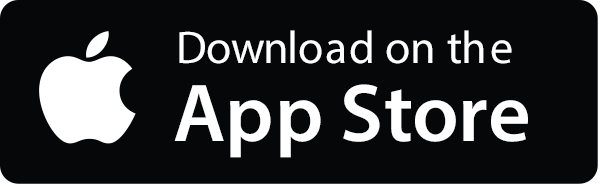
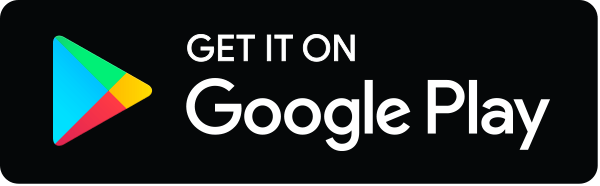