Objectives
- 1.
List the common principles that apply to all molecular motors: myosin, kinesin, and dynein.
- 2.
Describe the structure of a skeletal muscle cell and the organization of its contractile elements, and compare and contrast this with the structure of cardiac and smooth muscle.
- 3.
Describe the sliding filament mechanism of muscle contraction.
- 4.
Describe the coupling between the mechanical motions of the myosin motor and the steps involved in ATP hydrolysis during cross-bridge cycling.
- 5.
Describe how Ca 2+ interacts with the regulatory proteins troponin and tropomyosin to activate contraction in skeletal and cardiac muscle.
- 6.
Describe how Ca 2+ activates contraction in smooth muscle by promoting the phosphorylation of myosin regulatory light chain.
Molecular motors produce movement by converting chemical energy into kinetic energy
Movement is one of the defining characteristics of all living creatures. Motility is an essential feature of many biological activities, such as the beating of cilia and flagella, cell movement, cell division, development and maintenance of cell architecture, and muscle contraction, the main topic of this and the next two chapters. Indeed, the normal function of all cells requires the directional transport, within the cell, of numerous substances and organelles, such as vesicles, mitochondria, chromosomes, and macromolecules (e.g., mRNA and protein).
The three types of molecular motors are myosin, kinesin, and dynein
All types of cellular motility are driven by molecular motors that produce unidirectional movement along structural elements in the cell. The structural elements are either filaments composed of actin monomers or microtubules , which are polymers of the protein tubulin. Three distinct types of molecular motors that move along these structures have been described: myosin , kinesin , and dynein . Myosin is a motor that moves along actin filaments. There are many classes of myosins. Myosin II, which is found in all muscles, produces muscle contraction. Myosin V transports vesicles and organelles along actin filaments. Kinesin and dynein transport organelles along microtubules. Kinesins are also involved in spindle formation and chromosome separation during mitosis and meiosis, as well as in mRNA and protein transport. Dyneins mediate the beating of cilia, the movement of flagella, and vesicular trafficking.
Several principles apply to the operation of all molecular motors. Molecular motors convert chemical energy into kinetic energy (movement). The chemical energy is stored in the high-energy phosphate bond of ATP. The motors (myosin, kinesin, and dynein) all have ATPase activity. The binding of ATP, its hydrolysis, and the subsequent release of products are important steps in the generation of movement. In all cases, movement is produced through repetitive cycles of interaction between the motor and either an actin filament or a microtubule. The mechanism whereby ATP hydrolysis is coupled to the conformational and structural changes that produce movement has been elucidated through extensive biochemical, biophysical, and structural studies of muscle contraction and kinesin-based vesicle transport. The mechanism is discussed in a later section.
Single skeletal muscle fibers are composed of many myofibrils
Muscle cell types are classified primarily according to their structural and functional properties. An understanding of the detailed ultrastructure of single muscle cells provides insight into their functional properties. Skeletal muscle cells (skeletal myocytes) are attached to the skeleton by tendons and are under voluntary control. Their primary function is to shorten and generate force to produce movement of skeletal levers. The other two types of muscle, cardiac and smooth, are described later in this chapter.
Skeletal muscle is composed of many individual muscle fibers , each of which is an elongated cell. Each cell is 10 to 100 μm in diameter and may reach several centimeters in length. Electron micrographs reveal that a single skeletal muscle fiber is composed of bundles of filaments, called myofibrils . The myofibrils lie parallel to one another and run along the long axis of the cell ( Fig. 14.1 ). Surrounding each myofibril is an extensive membrane-enclosed intracellular compartment called the SR , which plays a key role in activating muscle contraction. Enlarged portions of the SR, the terminal cisterns, are closely apposed to finger-like invaginations of the sarcolemma (muscle PM) called transverse tubules (T-tubules) ( Fig. 14.1 ). The T-tubule membrane is continuous with the surface membrane. In contrast, the SR membrane is physically distinct, and electrically isolated, from the sarcolemma. The relevance of this point will become clear when we consider the roles of the T-tubule and the SR in excitation-contraction coupling in Chapter 15 . Viewed perpendicular to its long axis, a skeletal muscle cell has a striped appearance, with alternating light and dark bands ( Fig. 14.2 ); this has led to its classification as striated muscle .


The sarcomere is the basic unit of contraction in skeletal muscle
Sarcomeres consist of interdigitating thin and thick filaments
The banding pattern in striated muscle is produced by the regular arrangement of thick and thin filaments in the myofibrils. The light bands are I bands, which contain thin (actin) filaments that extend in both directions from a thin dense line, called the Z line ( Fig. 14.3 ). The region of myofibril between two adjacent Z lines is called a sarcomere . The dark bands, called A bands, contain thick (myosin) filaments arranged in parallel ( Fig. 14.3 ). At the center of the A band is a dense line called the M line. The thin filaments extend into the A bands, but are not present in the central H zone, a
The darker bands are called A bands because they are anisotropic; the I bands are isotropic. Anisotropic material has different refractive indices for different planes of polarized light; isotropic material has a single refractive index. The Z line takes its name from the first letter of Zwischenscheibe (intervening disk, in German). The H in H zone stands for heller (lighter, in German).
which therefore appears lighter. The regular arrangement of thick and thin filaments is clearly shown in a cross section of a myofibril taken in the region of the A band where the filaments overlap ( Fig. 14.3 ). The thick filaments interdigitate with thin filaments so that each thick filament is surrounded by a hexagonal array of thin filaments. This precise filament geometry is maintained by various cytoskeletal proteins that link filaments within a sarcomere and also link the sarcomeres of adjacent myofibrils. One of these important cytoskeletal proteins, α-actinin , is a major component of the Z line structure to which the thin filaments attach. Titin is a giant muscle protein (∼3.8 million daltons) that has an important role in muscle elasticity ( Chapter 16 ). One end of the titin molecule is inserted into the Z line; the other end forms a portion of the thick filament and inserts into the M line.
Thick filaments are composed mostly of myosin
With a molecular weight of approximately 470,000 daltons, myosin II is a large protein consisting of two heavy chains and two pairs of different light chains—a myosin essential light chain and a myosin regulatory light chain (RLC) . The myosin molecule has a long, rodlike tail with two globular heads ( Fig. 14.4 ). The rodlike portion of the molecule contains an “arm” adjacent to each globular head. At each end of the arm is a flexible region that acts as a hinge, allowing rotation at that point. Many myosin molecules align to form a thick filament ( Fig. 14.5 ). The tail regions of the molecules are bundled to form the body of the thick filament. The globular heads and arm regions project out from the bundle. The heads of the myosin molecules can bind to the thin filaments to form cross-bridges between the two filaments. The myosin heads in each half of the thick filament are oriented in opposite directions; the heads are not present in the central region ( Fig. 14.5 ).

Thin filaments in skeletal muscle are composed of four major proteins: Actin, tropomyosin, troponin, and nebulin
Actin is a globular protein (G-actin) with a molecular weight of 41,700 daltons. G-actin monomers aggregate to form strands resembling a string of pearls. The thin filament consists primarily of two helical strands of G-actin wound around each other ( Fig. 14.6 ). The 600-kDa protein molecule nebulin runs along the thin filament and forms a template that limits the length of the actin filaments. The thin filament also contains the regulatory proteins tropomyosin and troponin . Tropomyosin is a long, rod-shaped protein dimer with a molecular weight of approximately 66 kDa. This molecule lies along both sides of the thin filament in grooves formed by the two strands of actin molecules ( Fig. 14.6 ). Each tropomyosin molecule binds to seven actin monomers in one of the strands. Troponin, which is bound to tropomyosin, is a complex of three proteins: troponin T (TnT) , troponin C (TnC) , and troponin I (TnI) . The roles of tropomyosin and troponin in the Ca 2+ -dependent regulation of skeletal muscle contraction are discussed later in this chapter.

Muscle contraction results from thick and thin filaments sliding past each other (the “sliding filament” mechanism)
The sliding filament mechanism of muscle contraction was deduced from the changes in striation pattern of skeletal muscle observed during contraction. Before contraction, a relatively wide I band and H zone are visible ( Fig. 14.7 A). When stimulated to contract, muscle shortening is accompanied by sarcomere shortening ( Fig. 14.7 B). After the muscle shortens, the width of the A band is unchanged, but the widths of the I band and H zone decrease. The change in sarcomere length results from a change in the degree of overlap between thick and thin filaments as they slide past one another. In a resting muscle, only partial overlap occurs between thick and thin filaments ( Fig. 14.8 A). The region of the thin filaments that does not overlap the thick filaments corresponds to the I band, whereas the region of the thick filaments that does not overlap the thin filaments constitutes the H zone. When the muscle shortens during a contraction, the region of overlap between thick and thin filaments increases ( Fig. 14.8 B). In this contracted state, the H zones and I bands are narrower, because the nonoverlapped portions of thick and thin filaments are both shorter. The A band corresponds to the entire length of the thick filament. Because the filament length is constant, the length of the A band remains constant during changes in muscle length.


In the region of filament overlap, short connections, or cross-bridges, project from the thick filaments toward the thin filaments ( Fig. 14.8 ). The molecular basis for filament sliding involves cross-bridge movement. The cross-bridges attach to and pull on the thin filaments to cause sliding of the thick and thin filaments past each other. This increases overlap between the filaments and shortens the sarcomere.
The cross-bridge cycle powers muscle contraction
Cross-bridge movement produces filament sliding in the following way ( Fig. 14.9 ): the myosin head attaches to an actin filament to form a cross-bridge. The head then rotates toward the myosin tail, thus pulling on the thin filament and causing it to move relative to the thick filament. The head detaches and rotates back to its original orientation, and the cycle can repeat. This mechanism is analogous to rowing a boat. The oar is dipped into and pulled through the water (myosin binding and rotation; the “power stroke”), the oar is pulled out of the water and pushed back to its original position (myosin detachment and “recocking” of the head), and a new stroke can begin. These mechanical steps are coupled to the hydrolysis of ATP, which is catalyzed by the myosin head during its interaction with actin (i.e., myosin is an ATPase). The cyclical sequence of steps ( Fig. 14.9 ), the cross-bridge cycle , is the mechanism by which the muscle cell uses chemical energy stored in ATP to generate force.
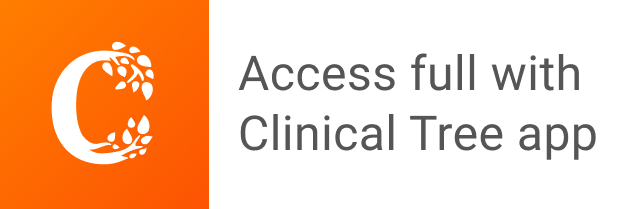