Fig. 10.1
This image shows the setup for MEPs and how the myogenic responses appear (Adapted with permission from Fig. 6, Schmidek and Sweet’s Neurosurgical Operative Techniques, 6th ed. Chap. 4. Intraoperative neurophysiology, pp 30–45. Elsevier, 2012. Original source: Deletis et al. [73]. This figure also adapted with permission from Pediatric Neurosurgery: Surgery of the Developing Nervous System. McLone D, ed. 4th ed. Chapter titled Intraoperative neurophysiological monitoring, pp 1204–1213. Elsevier 1999). (a) Schematic of electrode positions for transcranial electrical stimulation of the motor cortex. (b) Illustration of grid electrode overlying the motor cortex. (c) Recording of muscle motor-evoked potentials from the thenar and tibial anterior muscles
MEPs have a theoretical application to MI-LIF, but thus far have not been used for a number of reasons. First, usually only a single muscle group within the L5 or S1 distribution is monitored, creating a potential for false-negative readings at other spinal levels unless all myotomes are monitored. Second, due to deep positioning of the lower extremity motor areas within the interhemispheric fissure, a greater current is needed to obtain MEPs; it is often that the current is so high that the bilateral upper extremities are concurrently activated. A high-current intensity also creates nonspecific activation of the corticospinal tracts, including the internal capsule and brain stem/foramen magnum, whereas selectivity is only possible with small amounts of currents to specify discrete cortical locations. Normally the anode is the only stimulating electrode, but as the current increases, the cathode also becomes stimulating, creating bilateral activation [34]. Ultimately, the utility, ease, and accuracy of EMG make MEPs inefficient for use during MI-LIF.
10.4 Electromyography
EMG is being successfully used for other MIS procedures [35] and is the most commonly used method for motor monitoring during MI-LIF [6, 35–45]. EMG is advantageous for its ease of use and interpretation, especially during MI-LIF where nerves lie near the field of view and manipulation. As a test for the proximity of a nerve, it has a high sensitivity and low specificity, making it ideal for screening for nearby nerves [25]. Selecting the appropriate muscles for monitoring depends on the surgeon’s ability to access a specific myotome with a percutaneous needle, knowledge of anatomical safe zones during MI-LIF, and which specific nerves are at risk based on surgical anatomy [7–9].
For approaches to the lumbar spine, the nerve roots and distal nerves from T12 to L5 are at risk; it is important to note that even though EMG is normally used for monitoring motor nerves, spontaneous EMG can also be used with triggered EMG to detect antidromic stimulation of sensory nerves [45]. With these two thoughts in mind, Table 10.1 lists the specific nerves, the nerve roots at risk, and the recommended muscles or dermatomes for distal monitoring [43, 45–47].
Table 10.1
At risk nerves, their specific nerve root, and distal innervation
Nerve | Primary nerve root | Muscle |
---|---|---|
Subcostal | T12 | Rectus abdominis |
External oblique | ||
Iliohypogastric | T12–L1 | Transversus abdominis |
Ilioinguinal | Internal oblique | |
Genitofemoral (mainly sensory) | L1–L2 | Cremaster |
Scrotum (sensation) | ||
Labia majorum (sensation) | ||
Obturator | L2–L4 | Adductor longus/brevis |
Gracilis | ||
Adductor magnus | ||
Obturator externus | ||
Lateral cutaneous (only sensory) | L2–L3 | Thigh sensation |
Femoral | L2–L4 | Iliopsoas |
Quadriceps femoris | ||
Sartorius | ||
Vastus lateralis | ||
Pectineus | ||
Sciatic/deep fibular | L5 | Tibialis anterior |
Free-running EMGs are active whenever a nerve root is compressed, irritated, or injured, which causes spontaneous firing of the nerve and activation of the corresponding myotome. Each morphologic EMG activity reading corresponds to a specific neurotonic discharge: spikes represent individual discharges, bursts represent brief bundles of discharges, train activity represents persistent regularly repeated discharge patterns, and neurotonic discharges represent persistent prolonged bursting. A single burst of activity that occurs during surgical manipulations identifies and localizes a specific nerve and does not usually cause any deficit. However, activity that is sustained over 2 s is alarming and should prompt the surgeon to stop whatever specific procedure is causing the sustained activity.
Triggered EMG allows the surgeon to probe specific tissues that are suspicious for containing a nerve. The stimulator tip is a bipolar probe, which sends current into the area being probed to potentially activate any local nerves. EMG thresholds less than 5 mA indicate direct contact, and 5–10 mA indicates close proximity [43, 45, 48]. If there is a myogenic response, the surgeon knows to avoid that specific area.
Both free-running and stimulated EMGs should be used in tandem during the dissection of an MI-LIF. Great care should be taken during dissection through the psoas muscle as well as during dilation, as these procedures involve blind manipulation of surrounding structures that may cause nerve injury. Particularly during these times, free-running EMG should be activated and triggered EMG used frequently to localize potential nerves. EMGs with discrete thresholds and directional orientations are the most ideal, as they provide proximity and location of the nerve to the surgeon’s working space [45, 47, 48].
10.4.1 EMG Limitations
While EMG is sensitive in localizing nerves, it is not perfect in preventing nerve injuries for many reasons. Sharp immediate transection through a nerve will produce an immediate permanent injury without any EMG readout [45]. Additionally, the stimulated nerve may still shift within the soft tissue and change its location, requiring frequent sampling to ensure safety [45]. Recent studies have shown that chronic nerve stretching during retraction may not only injure the nerve [49, 50] but also decrease the ability of EMG to detect the nerve as it will become less responsive with greater time of retraction [50]. The incidence of motor neurological deficit ranges from 0.7 to 33.6 % [36, 37, 41, 45, 46, 50–61], with reports of false negatives during EMG use [55]. Ultimately, a transient neuropraxia may just be a consequence of nerve manipulation, especially those nerves that are smaller in caliber [45]. A dexamethasone bolus may help decrease nerve injury during these routine retractions [47, 52]. EMG is unable to identify nerve fibers of the sympathetic nervous system [62].
10.5 Mechanomyography
Mechanomyography (MMG) is a relatively new intraoperative monitoring technology. The basis for MMG monitoring is the detection of the mechanical oscillations on the muscle surface, which occur during muscle contraction, and it is the complementary mechanical signal to the electrical activity detected during EMG [63, 64]. It is primarily used for the identification of muscle fiber typing, assessment of muscle force, evaluation of muscle fatigue, characterization of the muscle resonance frequency, and assessment of muscle contractile properties [63, 65]. Its application to MI-LIF surgery is similar to that of triggered EMG, where the surgeon uses a probe to stimulate areas of interest; if a nearby nerve is stimulated, the MMG sensor will detect the mechanical signal of muscle contraction, alerting the surgeon of a potentially dangerous area. MMG has a few advantages over EMG. First, MMG sensors are noninvasive stickers, and their placement for detection of signal does not need to be precise nor specific [66]. Second, MMG is a mechanical signal, and so it is not affected by changes in skin impedance, which may occur when the skin is wet (sweat, normal saline, etc.) [67].
Conclusions
The use of EMG monitoring has contributed to the substantial decrease in nerve injury after MI-LIF [52, 68–70], and its routine use is strongly recommended [43, 46, 71]. A recent systematic review and meta-analysis of MI-LIF show strong evidence for the use of nerve monitoring and low-strength evidence that approaches without neuromonitoring have an increased neural complication rate [72]. While knowledge of anatomical safe zones should assist in the approach during MI-LIF [7–9], it should not exclude the use of EMG monitoring which has potential safety benefits with little additional cost. It is important to note that even after diligent monitoring, some patients may awaken with mild and temporary hip flexion weakness; this weakness may not be due to direct nerve injury, but from splitting of the psoas muscle during the approach [13, 43, 45, 47, 52, 53, 56, 71].
References
1.
Stern MB. Early experience with percutaneous lateral discectomy. Clin Orthop Relat Res. 1989;238:50–5.
2.
Farny J, Drolet P, Girard M. Anatomy of the posterior approach to the lumbar plexus block. Can J Anaesth. 1994;41:480–5. doi:10.1007/BF03011541.CrossRefPubMed
3.
Mayer HM. A new microsurgical technique for minimally invasive anterior lumbar interbody fusion. Spine (Phila Pa 1976). 1997;22:691–9; discussion 700.CrossRef
4.
McAfee PC, Regan JJ, Geis WP, Fedder IL. Minimally invasive anterior retroperitoneal approach to the lumbar spine. Emphasis on the lateral BAK. Spine (Phila Pa 1976). 1998;23:1476–84.CrossRef
5.
Pimenta L, Diaz RC, Guerrero LG. Charite lumbar artificial disc retrieval: use of a lateral minimally invasive technique. Technical note. J Neurosurg Spine. 2006;5:556–61. doi:10.3171/spi.2006.5.6.556.CrossRefPubMed
6.
Ozgur BM, Aryan HE, Pimenta L, Taylor WR. Extreme Lateral Interbody Fusion (XLIF): a novel surgical technique for anterior lumbar interbody fusion. Spine J. 2006;6:435–43. doi:10.1016/j.spinee.2005.08.012.CrossRefPubMed
7.
Moro T, Kikuchi S, Konno S, Yaginuma H. An anatomic study of the lumbar plexus with respect to retroperitoneal endoscopic surgery. Spine (Phila Pa 1976). 2003;28:423–8. doi:10.1097/01.BRS.0000049226.87064.3B; discussion 427–8.
8.
Regev GJ, Chen L, Dhawan M, Lee YP, Garfin SR, Kim CW. Morphometric analysis of the ventral nerve roots and retroperitoneal vessels with respect to the minimally invasive lateral approach in normal and deformed spines. Spine (Phila Pa 1976). 2009;34:1330–5. doi:10.1097/BRS.0b013e3181a029e1.CrossRef
9.
Benglis DM, Vanni S, Levi AD. An anatomical study of the lumbosacral plexus as related to the minimally invasive transpsoas approach to the lumbar spine. J Neurosurg Spine. 2009;10:139–44. doi:10.3171/2008.10.spi08479.CrossRefPubMed
10.
Uribe JS, Arredondo N, Dakwar E, Vale FL. Defining the safe working zones using the minimally invasive lateral retroperitoneal transpsoas approach: an anatomical study. J Neurosurg Spine. 2010;13:260–6. doi:10.3171/2010.3.spine09766.CrossRefPubMed
11.
Park DK, Lee MJ, Lin EL, Singh K, An HS, Phillips FM. The relationship of intrapsoas nerves during a transpsoas approach to the lumbar spine: anatomic study. J Spinal Disord Tech. 2010;23:223–8. doi:10.1097/BSD.0b013e3181a9d540.CrossRefPubMed
12.
Dakwar E, Vale FL, Uribe JS. Trajectory of the main sensory and motor branches of the lumbar plexus outside the psoas muscle related to the lateral retroperitoneal transpsoas approach. J Neurosurg Spine. 2011;14:290–5. doi:10.3171/2010.10.SPINE10395.CrossRefPubMed
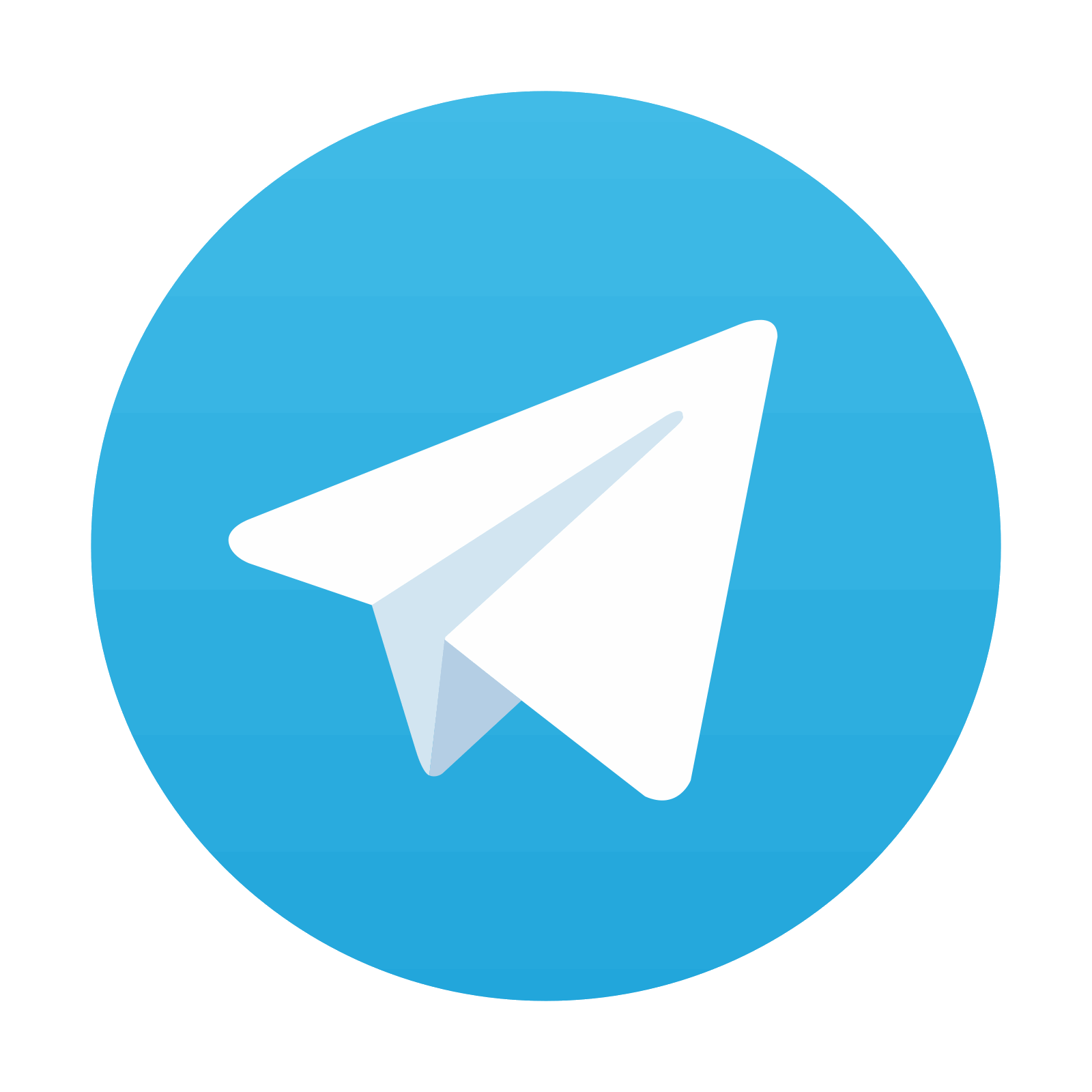
Stay updated, free articles. Join our Telegram channel
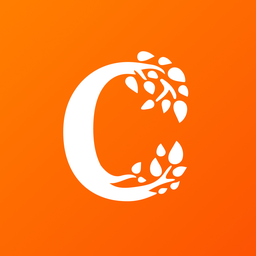
Full access? Get Clinical Tree
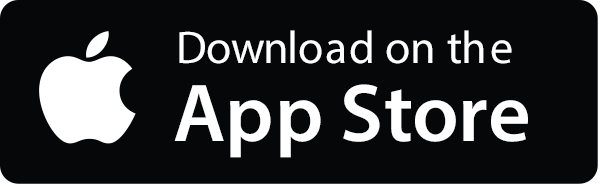
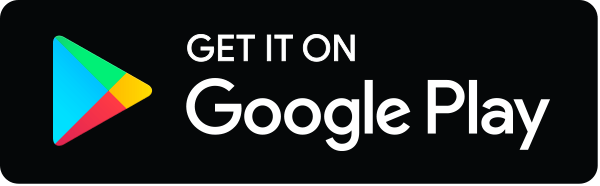